Department of Chemistry, Sant Gadge Baba Amravati University, Amravati, Maharashtra 444602, India
Received 13 November 2021; Revised 20 March 2022; Accepted 28 March 2022; Published 8 June 2022
Ashish Bansod, Ravindra Bhaskar, Chandarshekhar Ladole, Nilesh Salunkhe, Kanchan Thakare, and Anand Aswar, Synthesis, Characterization, Biological Activity and Solid-State Electrical Conductivity Study of Some Metal Complexes Involving Pyrazine-2-Carbohydrazone of 2-Hydroxyacetophenone, Journal of Transition Metal Complexes, 5 (2022), art246138. doi:10.32371/jtmc/246138
pyrazine-2-carbohydrazone; metal complexes; TGA; electrical conductivity; biological activity
Hydrazones are characterized by the presence of the triatomic grouping −C=N−N− and are found as interesting ligands in coordination chemistry due to their strong chelating ability through the electron delocalization, which is attached with extended conjugation, structure diversity and a wide range of possible applications [1,2,3]. Hydrazones and hydrazides have also gained considerable interest in recent years owing to their wide variety of biological and pharmacological properties as well [4,5]. Hydrazone Schiff bases continue to attract attention of several investigators due to their diverse biological applications like antimicrobial [6], antifungal [7], anticancer [8], herbicidal [9], and so forth. The metal complexes of hydrazones including heterocyclic moieties involving nitrogen, oxygen and sulphur as coordinating functionalities have been studied extensively in order to establish a relationship between the chemical structure and biological activity [10,11,12,13]. Earlier, we reported few metal complexes of biologically active hydrazone Schiff bases and compounds that showed interesting electrical and biological properties which prompted us to extend further our work with higher-valent metal ion complexes of pyrazine carbohydrazone of 2-hydroxyacetophenone to see metalation effect on such properties compared to substituted analog [14,15]. Considering the importance associated with pyrazine-2-carbohydrazide, in the present study we describe synthesis and characterization of Ti(III), Cr(III), Fe(III), WO2(VI), Th(IV) and UO2(VI) complexes with the ligand N′-(1-(2-hydroxyphenyl)ethylidene)pyrazine-2-carbohydrazide (H2L) derived from the condensation reaction of 1-(2-hydroxyphenyl)ethan-1-one with pyrazine-2-carbohydrazide (Scheme 1). After physicochemical characterization, these compounds were evaluated for their antibacterial and antifungal activities. Further, solidstate electrical conductivity of compounds has also been measured.
The chemicals used were all analytical reagent grade or chemically pure grade. Pyrazine-2 carboxylic acid and 1-(2-hydroxyphenyl)ethan-1-one (99%) (Aldrich Chemical Company, USA), acetylacetone, hydrazine hydrate (98%), anhydrous titanium chloride (99%), chromium chloride hexahydrate (96%), anhydrous ferric chloride (97%), thorium nitrate pentahydrate (99%), and uranyl nitrate hexahydrate (99%) were of analytical reagent grade, obtained from SD Fine Chemicals, Mumbai, India and were used as supplied (99% purity). Solvents employed were either of 99% purity or purified by known literature methods [16,17]. WO2(acac)2 was prepared according to literature methods [18].
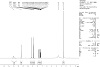
Figure 1: 1HNMR spectrum of H2L ligand.
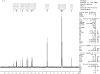
Figure 2: 13C NMR spectrum of H2L ligand.
Micro analyses of carbon, hydrogen, and nitrogen of the complexes were obtained using a Carlo Erba 1108 elemental analyzer. The IR spectra were recorded as KBr pellets using a Shimadzu 8201 spectrophotometer in the range 400–4000 cm−1. The metal and chloride contents were determined gravimetrically [15]. 1H and 13C NMR spectra of ligand were recorded on Bruker Advance II 400 MHz NMR spectrometer in DMSO-d6 using TMS as an internal standard. Magnetic measurements were carried out by the Sherwood magnetic susceptibility balance MK-1 at room temperature. The solid-state reflectance spectra of the complexes were recorded in the 200–1200 nm range using MgO as diluent on a Cary 60 UV-Vis spectrophotometer. Thermogravimetric analyses were performed on a Perkin Elmer, Diamond TG thermal analyzer in the temperature range 40–750 °C at a heating rate of 10 °C min−1 under a dynamic air atmosphere. X-ray diffraction patterns were obtained with a Bruker AXS, D8 Advance X-Ray diffractometer equipped with Si(Li) PDS. Mass spectrum of ligand was recorded on a Waters, Q-TOF micro mass (LC-MS) spectrometer. The surface morphology was observed using a JEOL Model JSM-6390LV scanning electron microscope. The solid-state electrical conductivity was measured as a function of temperature (313–373 K) in their compressed pellet form by using two probe methods. All the samples were compressed to pellets (13 mm dia × 1 mm) using a pressure of 3 ton cm−2. In order to provide a better electrical contact between pellet and electrodes, both surfaces of pellet were polished by silver paste and placed between two copper electrodes. The temperature of the sample was measured with the accuracy ±1 °C with a calibrated nichrome thermocouple inserted inside the cell. The activation energies of electrical conductance were calculated using Arrhenius equation σ = σ0 exp(Ea/KT).
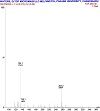
Figure 3: Mass spectrum of H2L ligand.
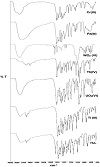
Figure 4: IR spectra of H2L ligand and its complexes.
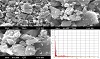
Figure 5: Scanning electron microscope images of (a) H2L ligand, (b) [Ti(L)(Cl)(H2O)2] complex, (c) [Cr(L)(Cl)(H2O)2] complex, (d) [Fe(Cl)(H2O)2HL2] complex, (e) [Th(L)(H2O)(NO3)2] complex, and (f) [UO2(L1)(CH3OH)] complex.
The ligand N′-(1-(2-hydroxyphenyl)ethylidene)pyrazine-2-carbohydrazide (H2L) was prepared according to the previously reported method [19]. The chemical structure of ligand was confirmed by various spectral characterizations (IR, 1H, 13C NMR, and mass spectra). The ligand has been synthesized under double stage synthesis and its synthetic scheme has been shown in Scheme 1.
Micro-analytical data for C13H12N4O2. Anal. Calc., C, 60.93; H, 4.72; N, 21.86. Found C, 61.12; H, 4.70; N, 21.83%. IR (KBr disc, cm1), 3,346 (OH), 3,182 (NH), 1,691 m(C=O), 1,630 m(C=N), 1,301 (C−O).
All the metal complexes [exceptWO2(VI)] were prepared in a similar way by the following general method. Equimolar quantities of metal salts (1 mmol) and ligand (H2L) (2.57 g, 1 mmol) were dissolved separately in DCM + MeOH (50:50 v/v) (∼ 25 mL) and both solutions were filtered and mixed in warm condition. The reaction mixture was refluxed for 5–6 h on a water bath. The pH of the reaction mixture was adjusted ∼ 6–7 by adding methanolic solution of sodium acetate (∼ 0.5 g) and refluxed further for another 1 h and then cooled to the room temperature. The solid product in each case was filtered off; washed several times with cold DCM, methanol, and petroleum ether; and dried under vacuum over CaCl2. Yield, 65–68%.
A hot solution of [WO2(acac)2] (0.34 g, 1 mmol) in ∼25 mL of methanol was added dropwise to a methanol (25 mL) solution of N′-(1-(2-hydroxyphenyl)ethylidene)pyrazine-2-carbohydrazide (H2L) (2.57 g,1 mmol) with continuous vigorous shaking. The resulting turbid solution obtained was filtered and then further refluxed on a water bath for 6 h. After reducing volume of the solution to Ca 10 mL and cooling to 10 °C overnight, the separated colored solid was filtered, washed with methanol followed by petroleum ether, and finally dried in desiccator over silica gel. Yield, 67%.
The Schiff base ligand (H2L) and its complexes were screened for their anti-bacterial and anti-fungal activity against E. coli MTCC 443, P. aeruginosa MTCC 424, S. aureus MTCC 96, B. subtilis MTCC 8979, E. faecalis MTCC 439, S. pyogenes MTCC 442, and fungal strains such as C. albicans MTCC 227, A. niger MTCC 282, and A. clavatus MTCC 1323 by using disc-agar diffusion method [20]. The solutions of ciprofloxacin (antibacterial drug) and clotrimazole (antifungal drug) were used as standard. Minimum inhibitory concentrations (MICs) of the compounds against test organisms were determined by the broth microdilution method [21,22,23,24] and DMSO was used as a negative control. All these tests were performed three times under identical conditions and average values were recorded. Activity was determined by measuring the diameter of zone showing complete inhibition and has been expressed in mm.
The reaction between pyrazine-2-carbohydrazide and 1-(2-hydroxyphenyl) ethan-1-one in ethanol yields a new hydrazone Schiff base ligand (H2L) [19]. The formation of ligand was confirmed by elemental and spectral data. The 1HNMR spectrum of ligand exhibits two resonances at 11.43 ppm and 12.99 ppm (singlet, 1H each) due to NH and phenolic protons, respectively. A sharp signal observed at 2.54 ppm due to the methyl protons of the hydroxyl acetophenone moiety indicates the formation of ligands. The mass spectrum of ligand showed a molecular ion peak at m/z = 257 which is consistent with its formula weight. Reaction of this ligand with metal salts resulted in the formation of complexes with good yield. All complexes are colored, stable at room temperature, and insoluble in common solvents such as ethanol, methanol, chloroform, benzene, cyclohexane, acetone and diethyl ether, but sparingly soluble in DMF and DMSO only. The elemental analyses suggest 1:1 metal–ligand stoichiometry for all the complexes and it is in good agreement with the proposed structures and geometry of complexes (Table 1). The insufficient solubility of the complexes, even in DMSO-d6, has prevented us from carrying out 1H NMR and UV-Visible solution studies of complexes.
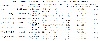
Table 1: Physicochemical and dc electrical conductivity data of ligand and its metal complexes.
The IR spectra of complexes are compared with that of the free ligand (H2L) to find out the points of attachment of the ligand to the metal ions in their complexes and assigning the coordination mode. When compared to free ligand, its characteristic IR stretching frequencies of imine and carbonyl groups are shifted on complex formation. The characteristic ligand bands at 1,691 cm−1, 3,182 cm−1 and 3,346 cm−1 due to C=O, NH and OH stretches respectively are not observed in the corresponding complexes, indicating the transformation of C=O and NH groups to the enolic form and their subsequent coordination to the metal ions through the deprotonation of phenolic and enolic hydrogen [25,26,27]. This has been further confirmed by the appearance of a new enolic band in the region 1,238–1,242 cm−1 due to ν(C−O) in the spectra of complexes. The enolization is favored by the stabilization of the anion by the conjugation with the > C=N−N=C < group. The ligand exhibits a strong band at around 1,630 cm−1 due to ν(C=N) (azomethine), and this band has been shifted to
lower frequencies in complexes by 31–33 cm−1, indicating donation of the lone pair of electrons on azomethine nitrogen to metal center [28]. This has been strengthened by the upward shift of ν(N−N) band from 977 cm−1 to 1,021–1,038 cm−1 it the spectra of complexes also supported the coordination of the azomethine nitrogen atom to metal ions [29]. The [UO2(L)(CH3OH)] complex exhibits bands at 900 (ν3) cm−1 and 867 (ν1) cm−1 assignable to νasy(O=U=O) and νsym(O=U=O) modes, respectively. The ν3 value has been used to calculate the force constant
(F) of ν(U=O) by the standard McGlynn et al. method [30].
The U−O bond distance is also calculated by the method of Jones [31]. The calculated FU−O and RU−O values are found to be 6.69 mdynes Å−1 and 1.74 Å respectively, showed
in the normal range for most of uranyl complexes. The ν(C−O) band of CH3OH is observed at 1,027 cm−1 and it showed a negative shift in UO2(VI) complex and is observed at 992 cm−1, indicating methanol is in coordination. The presence of a cis-WO2 moiety in the complex WO2(VI) may be inferred from the appearance of two bands in the 900–920 cm−1 region [32]. IR spectrum of Th(IV) complex shows two new non-ligand medium intensity bands at
1,425 cm−1 and 1,330 cm−1, due to coordinated nitrate ion. All complexes (except UO2(VI)) show broad bands at 3,338–3,432 cm−1, 1,541–1,579 cm−1 and 836–863 cm−1 due to ν(OH), δγ(H2O), and δw(H2O) vibrations for the coordinated water molecules [33]. Further, the appearance of new bands in the 527–568 cm−1 and 462–492 cm−1 regions for all the complexes could be attributed to the M−O and M−N, respectively.
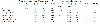
Table 2: Infrared spectral bands (cm−1) of the ligand and its metal complexes.
The reflectance spectrum of Ti(III) complex shows a broad band in the range 489–532 nm due to 2T2g → 2Eg, transition, in an octahedral symmetry around the Ti(III) ion [34]. The magnetic moment of Ti(III) complex is found to be 1.87 B.M. corresponding to one unpaired electron in an octahedral environment. The reflectance spectrum of Cr(III) complex shows three bands at 580 nm, 411 nm and 262 nm due to 4A2g(F) → 4T2g(F), 4A2g(F) → 4T1g(F), and 4A2g(F) → 4T2g(P), transitions, respectively, suggesting an octahedral environment around the chromium ion. The
magnetic moment value 3.97 B.M. is found within the acceptable range for the octahedral geometry.
The ligand field parameters: Dq Racah interelectronic repulsion parameter (B1), nephelauxetic ratio (β), and % covalency of the metal ligand bond, have been calculated by known relations [35]:
E[4A2g(F) → 4T2g(F) = 10 Dq
15 B1 = ν3 + ν2 − 3ν3
|
% covalency = (1 − β) × 100.
The calculated values of crystal field parameters Dq (1,724 cm1), B (709 cm1), β (0.77 cm−1) are also in good agreement with those reported for octahedral Cr(III) complexes (Table 3) [35]. The ν2/ν1 ratio is found to be 1.44 which is very close to the value of 1.42 obtained for most of the octahedral complexes. Electronic spectrum of Fe(III) complex shows three d-d bands at 833 nm, 528 nm, and 491 nm, which are assigned to 6A1g → 4T1g(G), 6A1g → 4T2g(G), and 6A1g → 4Eg(D) transitions, respectively, in octahedral geometry. The observed magnetic moment of 5.91 B.M. for Fe(III) complex is in the range expected for high spin octahedral system [36]. The Th(IV) and WO2(VI) complexes did not show any d-d transitions and are found to be diamagnetic as expected for their electronic configurations and likely to be an octahedral geometry. The electronic spectrum of UO2(VI) complex shows band at 492 nm due to the ligand to metal charge transfer transition. The UO2(VI) complex is found to be diamagnetic with coordination number six around the central metal ion.
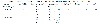
Table 3: Magnetic moments, electronic spectral data, and ligand field parameters of metal complexes.
The morphology and particle size of the Schiff base and its Ti(III), Cr(III), Fe(III), Th(III), and UO2(VI) complexes were characterized by scanning electron microscope (Figures 5(a)–5(e)) and revealed a presence of well-defined crystal structure free from any shadow of metal ion on their external surface. Ti(III) and Fe(III) complexes have stack of globule droplet-like structures while Cr(III), Fe(III), Th(III), and UO2(VI) complexes have platelet-like structures. The smaller average crystalline size found from XRD also shows that the particles are nanocrystalline phase.
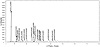
Figure 6: XRD diffractogram of ligand (H2L).
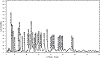
Figure 7: XRD diffractogram of [UO2(L)(CH3OH)] complex.
The XRD spectrum of H2L ligand (Figure 6) exhibits sharp peaks indicating the crystalline nature of the ligand. The 2θ values and the indexed X-ray diffraction data corresponding to the prominent peaks have been listed in Table 4. The unit cell data and crystal lattice parameters for the ligand (H2L) are found to be a = 10.3769 Å, b = 16.9788 Å, c = 6.7953 Å, α = 92.225°, β = 99.964°, γ = 93.369°, V = 1175.69 Å3 therefore the system is triclinic. X-ray powder pattern of UO2(VI) complex has been recorded as a representative case and the data are presented in Figure 7. X-ray crystal system has been worked out by trial and error method for finding the best fit between observed and calculated values and the data are given in Table 5. The unit cell parameters values obtained for [UO2(L)(CH3OH)] complex are system = triclinic a = 11.0807 Å, b = 13.2768 Å, c = 10.6823 Å, α = 113.506°, β = 104.982°, γ = 91.105°, V = 1,377.79 Å3.
The average crystallite size of the UO2(VI) complex was calculated from Scherer’s formula [37,38,39]. Using the full width at half maximum intensity of the pattern, the average size of the crystal is found to be 44 nm, indicating that the complex is in nanocrystalline phase.
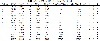
Table 4: Indexed X-ray diffraction data of H2L ligand.
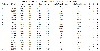
Table 5: Indexed X-ray diffraction data of [UO2(L1)(CH3OH)] complex.
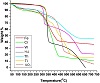
Figure 8: TG curve of ligand and its metal complexes.
Thermal analyses of ligand and its metal complexes were carried out in the temperature range 40–750 °C in air atmosphere with a heating rate of 10 °C min−1 to examine their thermal stability and to investigate the percentage mass loss of compounds. Thermograms are shown in Figure 8 and data is compiled in Table 6. The ligand decomposes in one step with continuous weight loss on increasing temperature with almost no residue at the end. The WO2(VI), Th(IV), and UO2(VI) complexes decomposed in two steps whereas Ti(III), Cr(III), and Fe(III) complexes decomposed in three steps. The elimination of coordinated water/methanol molecules takes place in first step in the temperature range 90–194 °C. The slight depression near temperature range 190–218 °C in Ti(II), Cr(III) and Fe(III) complexes after dehydration is due to the loss of coordinated chlorine molecule. After the loss of coordinated water/chloride ion, all the complexes show rapid degradation up to 750 °C. This may be due to the decomposition of organic part of the complex, indicated by the rapid fall in the percentage mass loss. A horizontal plateau on the TG curves for all the complexes indicates the decomposition of the complete organic part of the complexes in the last stage leaving residues as metallic oxide at the higher temperature range. From the TGA results, it is confirmed that the thermal stability of the complexes is higher than its free ligand and the complex is found to have formation of a five-membered chelate ring which may be attributed to the fact that the M−N and M−O bonds are highly polarized [40].
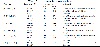
Table 6: Thermogravimetric data of metal complexes.
For the thermal degradation data of the complexes, various kinetic and thermodynamic parameters such as activation energy (Ea), frequency factor (Z), entropy (S), enthalpy of activation (ΔH), and Gibbs free energy (ΔG) were evaluated by employing the most popular Coats-Redfern relation [41] and values are given in Table 7. The parameters ΔH, ΔS, and ΔG were calculated by using the relations ΔH = Ea − RT, ΔS = [ln(Ah/kT) − 1], and ΔG = ΔH − TΔS, where k is Boltzmann’s constant and h is plank’s constant [42,43,44,45]. The reactions are classified on the basis whether they give off heat as exothermic (ΔH < 0) for absorb heat as endothermic (ΔH > 0). Further, the reactions are classified as exergonic (ΔG < 0) where the free energy of the system decreases and endergonic (ΔG > 0) where free energy of the system increases during the course of reaction. The higher value of activation energy of complexes indicates their thermal stability. The results revealed that the activation energy decreases with the increasing radius of metal cation. ΔG is positive for the reaction for which ΔH is positive and ΔS is negative. The reaction for which ΔG is positive and ΔS is negative reflects high thermal stability and unfavorable or nonspontaneous decomposition of the compounds. The estimated thermodynamic activation parameters are indicative of an endothermic process related to an irreversible thermal decomposition, suggesting a better molecular orientation in the activated state. The observed negative values of entropy for the decomposition reaction indicate a more ordered structure for the compound, and the decomposition reactions are slower than normal and also non-spontaneous in nature [46]. The correlations coefficients obtained for the thermal decompositions are lying in the range of 0.915 to 0.998, showing good fit with linear function.
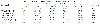
Table 7: Thermal decomposition data of ligand and its complexes.
The electrical conductivity (σ) of ligand and its complexes was measured as a function of temperature (T) in the temperature range 313–373 K in their compressed pellet form (3 ton cm−2). The values of electrical conductivities (σ) and activation energy (Ea) are listed in Table 1. The electrical conductivity (σ) varies exponentially with the absolute temperature according to the Arrhenius relation σ = σ°exp(−Ea/KT), where σ° is constant, Ea is the activation energy of electrical conduction, T is the absolute temperature, and K is the Boltzman constant. A linear dependence of logσ = f(103/T), as evident from Arrhenius plot of electrical conductivity (Figure 9), indicates the semiconducting behavior of these compounds [47]. The electrical conductivity value at room temperature lies in the range 7.32 × 10−12 to 7.34 × 10−10 Ω−1 cm−1 and activation energy 0.856–0.440 eV respectively. The observed low value of electrical conductivity of compounds may be attributed to low molecular weight due to which the extent of conjugation becomes low or undesirable morphology due to pressing of the sample into hard brittle pellet form.
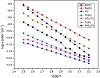
Figure 9: Temperature dependence of log σ.
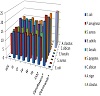
Figure 10: Antimicrobial activity of the H2L ligand and its metal complexes.
All the newly synthesized compounds were screened in vitro for their antibacterial and antifungal activities against bacterial strains S. aureus MTCC 96, S. pyogenes MTCC 442, B. subtilis MTCC 8979, E. coli MTCC 443, P. aeruginosa MTCC 424, and E. faecalis MTCC 439 and fungal stains A. niger MTCC 282, A. clavatus MTCC 1323, and C. albicans MTCC 227. The results were recorded for each tested compound as the average diameter of inhibition zones of bacterial growth surrounding the well in mm. The obtained results are presented in Table 8 and shown in Figure 10. It is clear that all of the metal complexes are more potent bactericides and fungicides compared to the free ligand (H2L). It is also observed from the data that the complexes are slightly more effective towards Gram-positive strains as compared to the Gram-negative ones, though the level of action of a particular compound within the same type of bacteria is varying. This difference in the activity probably may be attributed to the fact that the cell wall of Gram-positive bacteria have more antigenic properties as the outer lipid membrane is of polysaccharides. Although the complexes showed promising activities against the bacterial strains, however, their activities were found to be less than the standard ciprofloxacin (antibacterial drug) and clotrimazole (antifungal drug). This enhancement in the activity was suggested to be possibly due to an efficient diffusion of the metal complexes into the bacterial cells or interaction with the bacterial cell walls. The results reflect that Ti(III), Cr(III), and Fe(III) complexes exhibited higher activity, while Th(IV), WO2(VI) and UO2(VI) complexes showed moderate activity against all bacterial and fungal stains. This growing in the activity could be explained based on the chelation theory [48]. The chelation decreases the polarity of the metal extremely and fundamentally due to the partial involvement of its positive charge with donor groups and possible π-electron delocalization overall chelate rings. The lipid and polysaccharides are some important constituents of cell wall and membranes, which are favorable for metal ion interaction [49,50,51]. Chelation not only can diminish the polarity of the metal ion, but also can raise the lipophilic property of the chelate and the interaction between metal ions and the lipid is preferable. This could lead to the slump of the permeability barrier of the cell resulting in interference with normal cell processes. If the geometry and charge distribution around the molecule are incompatible with geometry and charge distribution around the pores of the bacterial cell wall, then penetration through the wall by the toxic agent cannot take place and this will prevent the toxic reaction within pores. Chelation is not the only criterion for the antimicrobial activity. Some important factors such as the nature of the metal ion, nature of the ligand, coordinating sites, geometry of complex, concentration, hydrophobicity, lipophilicity, and presence of co-ligands have considerable influence on antimicrobial activity. The higher activity of Ti(III), Cr(III), and Fe(III) complexes due to the presence of chlorine group in the structure of complex may be playing an important role towards activity. From the antimicrobial study, it is found that tested compounds possess better antimicrobial activities and also comparable to standards used.
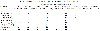
Table 8: Antimicrobial activity of the H2L ligand and its metal complexes.
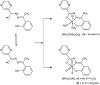
Figure 11: Schematic diagram for complexation processes.
The authors are thankful to Sophisticated Test & Instrumentation Centre, Cochin, Kerala for providing Powder XRD, TG-DTA, and SEM. Authors are also thankful to Sophisticated Analytical Instrument facility, Punjab University, Chandigarh for providing elemental analysis and IR, 1H NMR, and 13C NMR spectra facility. Sant Gadge Baba Amravati University, Amravati (Maharashtra) for providing laboratory facilities is also gratefully acknowledged.
The authors declare that they have no conflict of interest.
Copyright © 2022 Ashish Bansod et al. This is an open access article distributed under the terms of the Creative Commons Attribution License, which permits unrestricted use, distribution, and reproduction in any medium, provided the original work is properly cited.