Vol. 3 (2022), Article ID 246141, 10 pages
Research Article
Primary Chondrocyte Cilium Strains Increase with Depth Within the Growth Plate Reserve Zone and Reflect Loading Direction Across the Growth Plate
Paola M. Jimenez Carrion and John Leicester Williams
Biomedical Engineering Department, University of Memphis, 330 Engineering Technology Bldg, Memphis, TN 38152, USA
Received 2 December 2021; Revised 14 February 2022; Accepted 21 February 2022; Published 21 March 2022
Paola M. Jimenez Carrion and John Leicester Williams, Primary Chondrocyte Cilium Strains Increase with Depth Within the Growth Plate Reserve Zone and Reflect Loading Direction Across the Growth Plate, Mechanobiology Journal, 3 (2022), art246141. doi:10.32371/mbj/246141
Background. It is known that reserve zone (RZ) chondrocytes regulate the organization of the physis and that chondrocyte primary cilia (PC) act as mechanotransducers. Objective. To explore if physeal loading-induced strains in RZ chondrocyte PC can enable cells to sense whether the physis is subjected to increasing or decreasing levels of compression. Methods. Finite element models of RZ chondrocytes within the physis were created to examine strains developed in the PC when the physis is subjected to either 10% compression or tension. The PC were oriented either toward the epiphysis or metaphysis. Findings. PC basal body axial and transverse strains were negative when the physis was compressed and positive when subjected to tension. Axial strains in the cilium body transition zone also followed the applied loading and were amplified up to 4X, whereas membrane strains transverse to the cilium were tensile under physeal compression, possibly stretching integrin receptors. Tension applied to the physis stretched the cilium membrane in the axial direction. Conclusion. PC perceive strains in ways that may explain the regulatory function of RZ chondrocytes and their role in modulating bone growth.
cartilage; epiphyseal plate; bone development; cilia; mechanobiology
Growth plate chondrocytes moderate bone growth in response to mechanical loads by transducing strains and stresses into intracellular biochemical signals through a variety of mechanisms under active investigation. Computational models of cells in the proliferative zone (PZ), hypertrophic zone (HZ), and reserve zone (RZ) have revealed depth- and zone-dependent variations in cellular stress and strain [1,2,3,4,5]. Computational modeling has also shown that the RZ chondrocyte pericellular matrix (PCM) is an essential component of the cell’s mechanosensory mechanism [3]. Not included in these models is the primary cilium which is located in the PCM and has been shown to be an important mechanism for signal and force transduction [6] and necessary for cartilage development [7].
Ciliary function is required for normal growth. It has been shown that depleting cilia in mouse growth plate chondrocytes by disrupting intraflagellar transport deranged cell and column orientation and resulted in dwarfism [8]. Besides the presence or absence of cilia, their lengths also appear to be relevant to the cell’s mechanosensory mechanism as shown by the association of increased cilia lengths in osteoblast cells of patients with idiopathic scoliosis [9]. In addition to cilium length, cilium orientation is important for establishing the axis of bone growth. The primary cilia in the PZ and HZ are aligned parallel to the longitudinal axis of the bone, implying that polarization gradients exist inside the growth plate that direct the cells to their specific positions and orientations [10]. This polarity was shown to be disturbed but partly retained in growth plate sub-populations of small columns of osteochondroma cells [10]. Cilia do not appear to be polarized in the RZ but it has been suggested that the RZ cells secrete morphogens [10] that control the alignment of clones in preparation for the transition into the PZ [11]. The growth plate’s response to mechanical loads is mediated by the cilium through gene expression thereby affecting proliferation, differentiation, and organization of the growth plate [12]. Despite the apparent importance of the RZ as a regulator of the physis [13] and of primary cilia as mechanotranducers, no studies have examined the mechanical signals in and around cilia in the RZ.
In this study we used computational modeling to explore the following questions: (1) Do cilia perceive strains differently depending on cell depth in the RZ region between the subchondral epiphyseal bone plate and PZ in ways that could be associated with cell recruitment into the PZ? (2) Do cilia perceive strains differently when the growth plate is subjected to compression versus tension in ways that could explain modulation of bone growth by the Hueter-Volkmann principle? (3) Could the shear stress in the pericellular matrix be a factor in chondrocyte kinesis and rotation near the PZ?
A 2D axisymmetric, static finite element model of a central region of bone-growth plate-bone volume was created using ABAQUS/CAE 2019. A uniform displacement of 0.08 mm was prescribed on the surface of the sample in a direction perpendicular to the growth plate which equals 10% of the 0.8 mm growth plate cartilage thickness (−Y for compression and +Y for tension); see Figure 1. Axisymmetric 4-node bilinear, hybrid, constant pressure elements (CA4XH) were used to avoid volumetric locking due to the nearly incompressible material properties assigned to some of the regions (Table 1). Mesh convergence studies showed the model converged when the Von Misses stresses in the cell nucleus and cilium varied by less than 5% between consecutive refinements. Non-linear geometric effects and large strains were accounted for by turning on NLGEOM.
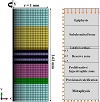
Figure 1: 2D plane axisymmetric model of a central cylindrical volume of bone-growth plate-bone. Nodes along the axis of symmetry (Y-axis) were free to move in the axial or Y-direction and were fixed in the radial or X-direction. Nodes along the bottom were fixed in the Y-direction and free in the X-direction. Nodes at X = 1 mm were unconstrained and nodes at the top were free in the X-direction. A displacement of 0.08 mm was applied in the + or −Y-direction along the top boundary. Cells were included at three locations in the RZ.
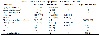
Table 1: Linear elastic material properties of the model components.
The growth plate was partitioned into two sections to represent the RZ and the PZ/HZ. Cells were embedded at three locations (Figure 2): Cell 1—close to the epiphyseal subchondral bone-plate border at the upper end of the RZ, Cell 2—in the middle, and Cell 3—at the lowest level near the PZ border. Each cell was surrounded by a PCM, cell membrane, cilium, and ciliary membrane; and was composed of a cytosol and nucleus. Isotropic linear elastic material properties and dimensions for the various regions in the model were obtained from the literature (Table 1). The primary cilium had a radius of 0.1 μm and length of 2.5 μm (0.5 μm inside the cell and 2 μm in the PCM). All regions of the cilium body were assigned a Young’s modulus (YM) of 178 kPa, Poisson’s ratio (PR) of 0.33. The cilium was surrounded by a membrane of 10 nm thickness.
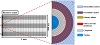
Figure 2: Enlarged view of the RZ region of the idealized axisymmetric model shown in
Figure 1. Three chondrons were in the RZ and each consisted of a PCM, a primary cilium with a ciliary membrane, a cell membrane, a cytoplasm, and a nucleus, as shown in the detail view for Cell 2 in the middle of the RZ.
Two orientations of the cilium were considered, both aligned with the long axis of the bone in the major direction of bone growth: oriented either towards the epiphysis or the metaphysis. To describe the results the cilium was segmented into several regions: basal body, transition zone, proximal axoneme, middle axoneme, and distal axoneme. The basal body extended 0.5 μm inside the cell beneath the cell membrane, the transition zone was at the level of the cell membrane, and the proximal, middle, and distal axoneme regions projected 2 μm into the PCM with the distal end of the cilium located 0.5 μm from the outer radius of the PCM. The ciliary membrane covered the portion of the cilium extending into the PCM and contained three regions: proximal, middle, and distal ciliary membranes. The centroidal value for the elements in each cilium and cilium membrane region was used to report axial (Y-) and transverse (X-) strains.
Axisymmetry prevents consideration of other orientations in which bending would play a role in deforming the cilium. To investigate stress conditions within the PCM at other possible cilium orientations, in-plane shear stresses and maximum shear stress contour plots were obtained within the PCM. Shear stress values at 0, 90, and 180 degrees to the Y-axis were plotted at the location where the distal end of the cilium would be if it were included in the PCM (at a radius of 7 μm, 2 μm out from the cell membrane, and 0.5 μm away from the PCM/ECM interface).
To investigate the sensitivity of the cilium strain results to the assumed material property values for the cytoplasm we increased the cytoplasm’s YM ten-fold from 3.5 kPa to 35 kPa. This increase may also represent various states of cytoskeletal activity in response to growth plate loading. The cell height and width strains were calculated from the ratios of the changes in the vertical cell diameter and radius before and after compression to the undeformed values. All other strains were reported as logarithmic strains.
Cilium strains varied with the depth location of the chondrocyte within the RZ (Figure 3). At 10% applied compression cilium axial strains were compressive, doubling in magnitude near the bottom of the RZ and amplifying axial strains nearly 4X within the cilium transition zone and amplifying compressive transverse strains 2X within the basal body region (Cell 3). Cilium transverse strains outside of the cell body became less compressive with increasing RZ depth and became tensile near the PZ (Cell 3). Cilium membrane strains also varied with cell location and were compressive in the axial direction, doubling in magnitude near the PZ border (Cell 3). Cilium transverse membrane strains were compressive for the cell at the top of the RZ (Cell 1), but tensile in the middle (Cell 2) as well as at the bottom of the RZ (Figure 4).
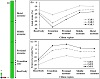
Figure 3: Cilium strains in RZ chondrocytes at 10% compression. (a) Representation of cilium regions for reporting strains; (b) Depending on the depth of the cell within the RZ the cilium axial (Y-) strains were amplified 2–4X within the cilium transition zone and became more compressive with RZ depth. (b); (c) Cilium strains were amplified 1.25–2X within the basal body region in the transverse (X-) direction and became less compressive with increasing RZ depth and even tensile in more distal regions of the cilium at the deepest location near the PZ (Cell 3).
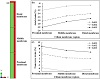
Figure 4: Cilium membrane strains in RZ chondrocytes at 10% compression. (a) Representation of cilium membrane regions. (b) Axial (Y-) membrane strains were compressive and increased in magnitude with the depth of the cell in the RZ, reaching 2X the applied strain in the cilium proximal membrane region for the cell near the PZ (Cell 3). (c) Transverse to the membrane (X-) strains were tensile for the cell near the PZ (Cell 3) and in the middle of the RZ (Cell 2), but compressive for the cell near the subchondral epiphyseal bone plate. Transverse membrane strain magnitudes decreased distally along the length of the cilium.
Cilium strain patterns reflected the direction of loading. Compared with 10% compression, simulating 10% distraction generated an inverted pattern of strains along the cilium compared at all depth locations. Axial strains reflected the sense of the applied load vector, whereas transverse strains reflected the opposite sense (Figure 5). The results were identical whether the cilium was placed at 180 or 0 degrees to the +Y-axis, demonstrating no apparent up or down preference in terms of strain sensitivity for the cilium in the RZ when aligned with the axis of bone growth.
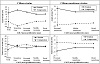
Figure 5: Cilium and cilium membrane strains in Cell 3 (cell near the PZ) for a growth plate subjected to either a 10% tensile or a 10% compressive strain. Cilium axial strains were amplified 3–4X within the cilium transition zone and 2X within the basal body region. Cilium membrane strains were amplified 2X proximally along the cilium axis. Strains transverse to the cilium membrane were tensile for compressive loading and compressive for tensile loading.
The in-plane shear stresses in the PCM increased 5-fold with cell depth at 0 and 180 degrees from the positive Y-axis
and at a radius corresponding to the cilium distal end, but only increased slightly at 90 degrees. Near the cell surface the in-plane shear stresses were concentrated at 45 and 135 degrees (contour plots in Figure 6(a)) increasing from 8 kPa for Cell 1 to 13 kPa for Cell 3. The corresponding maximum 3D shear stresses increased in magnitude with cell depth within the RZ from 15 kPa for Cell 1 to 40 kPa for Cell 3 at 0 and 180 degrees, and from 14 kPa to 46 kPa at 90 degrees. At the cell membrane surface, the maximum 3D shear stress values were concentrated at the 0- and 180-degree locations, doubling in value with cell depth from 31 kPa for Cell 1 to 69 kPa for Cell 3 near the PZ and reaching local peaks of 45 kPa to 55 kPa at 90 degrees for Cells 2 and 3, respectively (Figure 6(b)).
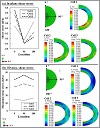
Figure 6: Line graphs and contour plots of (a) in-plane shear stress and (b) 3D maximum (Tresca) shear stress within the PCM of cells in three locations in the RZ when subjected to 10% compression of growth plate cartilage. In-plane shear stresses and maximum shear stresses in the line plots were extracted at a radius of 7 μm corresponding to the distal tip of the cilium at three locations: 0, 90, and 180 degrees. Contour plots are in units of MPa. Cell 1 is located near the subchondral bone plate. Cell 2 is in the middle of RZ and Cell 3 is near the PZ. The outer radius of the PCM is 7.5 μm and the inner radius of the PCM is 5 μm.
The cilium strain results were insensitive to a 10% increase in YM of the cytoplasm or the nucleus. A ten-fold increase in YM of the cytoplasm had a negligible effect on chondrocyte height and width strains and relatively small changes were seen for the nucleus strains which became more pronounced near the bottom of the RZ. For Cell 3 increasing the cytoplasm modulus caused the nucleus width strain to change from 9% to 13%, and the height strain to change from −17% to −22% (Figure 7). Cilium strains were not affected by increases in YM of the cytoplasm.
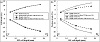
Figure 7: Plots showing the influence of a 10-fold increase in YM of the cytoplasm on cell and nucleus height and width strains at 10% compression in three cell locations in the RZ: near the subchondral bone plate (Cell 1), in the middle of RZ (Cell 2), and near PZ the (Cell 3). (a) Chondrocyte height and width strain at each cell location. (b) Nucleus height and width strain for each cell location. The influence of the cytoplasm modulus on cell nucleus strain is more pronounced as the cell location approaches the PZ. Cilium strains were unchanged by the increase in cytoplasm modulus.
A model was developed to investigate the possible role of the chondrocyte primary cilium in transducing mechanical signals in the growth plate RZ. We explored the sensitivity of ciliary strains for chondrocytes located at increasing depths within the RZ from the top near the subchondral bone plate (Cell 1) to the bottom near the PZ borders (Cell 3); Ciliary strains are especially of interest for understanding their possible role at the top of the PZ, where the cells divide and rotate to form a column of proliferating cells. We also examined the sensitivity of ciliary strains to changes in loading direction by considering applied tensile versus compressive loading. Understanding how the cilium strains develop under these different loading scenarios may provide insight into how loading modulates growth. Lastly, we examined the relationship between pericellular matrix shear stresses and possible ciliary orientations in the RZ, noting that studies have shown no preferred ciliary orientation for chondrocytes in the RZ other than when the cells enter the columnar zone where the cilia orient with the columnar axis (0 and 180 degrees to the Y-axis in the model).
Compression of the growth plate by 10% produced the highest compressive strains (40%) in the transition zone of the cilium in the RZ cell closest to the PZ. High strains have also been reported in this transition region of the cilium for other tissues subjected to other loading environments [23,24]. These high strain levels in the cilium transition zone hint at the mechanosensory role of the cilium not only through transmembrane channels present in the base of the cilium, but also through bridging proteins such as integrins that help the cilium bind with the PCM or cytoskeleton. The transition zone of the cilium is known for accumulating and filtering the entry of multiple proteins including transport motor proteins that run along the long axis of the axoneme [25,26]. Thus, the high concentration of molecules and proteins in the proximal axoneme might be related to the high strains developed in the proximal membrane.
Strains in the transverse direction inside the cilium and across the ciliary membrane became tensile and close in magnitude to the applied 10% compressive strain in RZ cells at the border of the PZ, which has half the elastic modulus of the RZ, but remained compressive near the calcified cartilage of the subchondral bone-plate, which has a modulus 300 times greater than the RZ. At the PZ border, these tensile strains acting transverse to the cilium membrane are likely transmitted from the PCM to the cilium through integrins attaching the cell and cilium to the PCM. RZ chondrocytes at the PZ transition organize themselves to enter PZ columns through cell division and rotation [10,27]. Previous studies [8,28,29] have linked the primary cilia to cell differentiation and signaling factors which stimulate the proliferation, differentiation, and rotation of the chondrocyte as it enters the PZ. In addition, the transition zone of the cilium, also known as the “check-point,” is the location where most of the proteins are being regulated and sorted [25,29]. Thus, the tensile transverse strains across the entire length of the cilium membrane, that are maximum at its transition zone, may stimulate the organization of cell cytoskeleton to prepare for kinesis, rotation, and entry into the PZ tubular columns.
Both compression and tension produced a notable depth-dependent response in chondrocyte cilium strains suggesting that chondrocytes sense external loading through the primary cilium differently depending on their position in relation to the chondron-osseus border and the PZ border. Tension across the growth plate produced a similar pattern of depth-dependence in axial cilium and membrane strains as was seen for compression, except that the strains were
reversed in sign. Under applied tension the axial cilium membrane strains in the cell near the PZ were tensile and transverse strains were compressive, whereas under applied compression the axial cilium membrane strains were compressive and the membrane transverse strains were tensile. In both tension and compression, the primary cilium strains for cells close to the PZ were larger than for cells near the subchondral bone-plate. In both cases the largest axial strains occurred in the transition zone of the cilium and in the proximal cilium membrane; thus, applying compression or tension to the growth plate caused the most changes where the cells are transitioning from the RZ to the PZ.
As to whether cells can distinguish loading by compression from tension by means of the cilium, it may be noted that both the axial and transverse strains in the cilium basal body of the cell near the PZ follow the applied loading sign; i.e., growth plate compression produced compressive axial and transverse strains, while tension produced tensile axial and transverse strains in the basal body. These results suggest ways by which cilia could distinguish compression
from tension. The basal body plays a role in motility and cell cycle progression as well as morphogenesis. It is possible that tension and compression of the basal body could initiate different biological responses. Another possibility is that tension across the growth plate stretches the proximal cilium membrane thereby opening stretch activated ion channels, while compressive loading results in tensile strains in the direction transverse to the membrane/PCM interface and stretches integrin receptors [30]. Each of these events may lead to different cellular responses that modulate bone growth.
We found no differences in cilium strains between the 0- and 180-degree orientations of cilia in the present study. The orientation of the cilium has been noted to be disrupted in cartilage diseases such as osteoarthritis, osteochondroma, and chondrosarcoma [10,31,32,33]. In the PZ and HZ of growth plate cartilage, primary cilia are present at the top or bottom of the cell and oriented towards the subchondral bone or towards the metaphysis. In the RZ, however, no clear pattern for orientation has been determined [10]. It has been proposed that cilia are oriented in a particular direction partly for protection of the cilium itself as well as preserving intracellular structures [31,33,34].
In this study, the pericellular matrix shear stresses were concentrated in three locations along the cell surface and were greatest at the 0-, 90-, and 180-degrees locations indicating that a primary cilium located there will experience more shear stress at the cell membrane junction than at other locations. The maximum matrix tissue shear stresses of 31 kPa to 69 kPa in the present study are comparable to the 55 kPa value reported for articular cartilage under 10% compression [35]. These maximum shear stresses in the PCM increased in magnitude with cell depth, and peak values were seen for the cell near the PZ, where cells are positioned to join a column or divide and rotate to form daughter cells and orient their cilia vertically in the PZ. It has been shown that the primary cilia are non-polarized in the RZ but become polarized and orient parallel to the longitudinal axis of the bone in the PZ and HZ [10], coinciding with regions where we found peak maximum shear stresses. The shear stresses are of interest because they could cause stretching of integrins anchoring the cell and cilium to the PCM and stimulate cell kinesis and rotation, which are likely to be involved as RZ cells’ transition from RZ to PZ.
In the present model the primary cilium strains and the cell height and width strains were insensitive to a 10-fold increase in YM for the cytoplasm, while nucleus strains showed some sensitivity suggesting that the cytoskeleton plays a role in transmitting strains to the nucleus, perhaps in connection with signals from the primary cilium [36]. For compression of the growth plate by 10%, cell strains increased with cell depth within the 0.4 mm thick RZ reaching maximum values of −20% height strain and 11% width strain for the chondrocyte at the columnar zone border. This trend is the same as shown in a previous study on the RZ [3] in which the RZ thickness was 0.95 mm and the cell strain values at 0.4 mm depth were −25% for height strain and 15% for width strain when the growth plate was compressed by 15%.
The model represents a 2-mm diameter cylindrical volume of tissue from an interior region of a growth plate in which the outer circumferential surface of the model is far enough from the axis where the cells were located, for boundary conditions to not affect the local stress field around the cells, as was determined by comparing various diameter models. The thickness values of each growth plate zone were chosen based on histology sections of the proximal femoral epiphysis of 480-day-old pigs [4]. The RZ thickness was half that of the previous study while the other zones had similar values. This range in values is representative of what is seen in the histological sections. The model is nonlinear and accounts for large deformation but assumes linear elastic material behavior. We employed a single phase, isotropic, elastic model to avoid the complexity of the multiphase, anisotropic, and non-homogeneous properties since those have not been determined for RZ cartilage in the growth plate and are not well characterized for the proliferative and hypertrophic layers. While representing cartilage as a single-phase material is a significant approximation of cartilage behavior, we argue that it can serve as a reasonable approximation of either the long-time equilibrium response [37] or the shorttime behavior [5]. Based on comparisons between a multiscale poroelastic model at high strain rates [5] and an elastic model of cells embedded within the growth plate RZ [3], we believe that a single-phase elastic model provides insight into the mechanical environment within the growth plate for loading at high strain rates during short time intervals such as during initial foot and ground contact in gait or running.
Epiphysial plates contribute to most of the longitudinal growth of a long bone. These cartilaginous joints lie at right angles to the principal compressive stress directions and are mainly subjected to compressive loading and to some shear loads [38]. It is conceivable and perhaps even likely that portions of the epiphyseal plate are subjected to short periods of tensile loads across the plate such as would occur during the application of bending moments across a joint. One situation requiring consideration of tension is the clinical treatment for limb lengthening known as physeal distraction or chondrodiatasis [39] in which the growth plate is stretched by 0.25 mm twice daily. Tension across the growth plate may also have relevance to a new treatment for scoliosis in which vertebral bodies are tethered [40] so as to slow vertical growth on the convex side of the vertebrae by tensioning of the tether, while unloading and perhaps inducing some tension across the growth plate on the concave side.
The idealized model of the cilium, represented by four elements across its thickness, did not include fibers that form a bridge between the transition zone microtubules and the ciliary membrane [24] or the nine pair-ring structure of the axoneme which is composed of microtubules [41] which are likely to be important to understanding the mechanobiology of the cilium. More detailed models are required to further explore the connection to the intracellular components and cytoskeletal structure. The results of this idealized axisymmetric model suggest that further work is warranted with a full 3D model to explore other ciliary orientations besides those limited to be vertical, but to our knowledge, this axisymmetric model is the first model to include the primary cilium, cell membrane, nucleus, cytoplasm, pericellular matrix, and relevant growth plate tissue components to examine the stress-strain behavior of a chondrocyte under tension and compression in the appropriate in situ environment.
This research was funded by the University of Memphis.
P. M. Jimenez Carrion conducted the simulations. Both authors contributed to the manuscript writing and approved the final text.
The authors declare that they have no conflict of interest.
- J. Gao, E. Roan, and J. L. Williams, Regional variations in growth plate chondrocyte deformation as predicted by three-dimensional multi-scale simulations, PLoS One, 10 (2015), e0124862.
- J. Gao, J. L. Williams, and E. Roan, Multiscale modeling of growth plate cartilage mechanobiology, Biomech Model Mechanobiol, 16 (2017), 667–679.
- M. Kazemi and J. L. Williams, Chondrocyte and pericellular matrix deformation and strain in the growth plate cartilage reserve zone under compressive loading, in Computer Methods, Imaging and Visualization in Biomechanics and Biomedical Engineering. CMBBE 2019, G. Ateshian, K. Myers, and J. Tavares, eds., vol. 36 of Lecture Notes in Computational Vision and Biomechanics, Springer-Verlag, Cham, 2020.
- M. Kazemi and J. L.Williams, On the role of the reserve zone and mechano-regulatory stimuli in the development and maturation of the growth plate: observations and models, Mechanobiol J, 2 (2021), art246116.
- M. Kazemi and J. L. Williams, Depth and strain rate-dependent mechanical response of chondrocytes in reserve zone cartilage subjected to compressive loading, Biomech Model Mechanobiol, 20 (2021), 1477–1493.
- R. Ruhlen and K. Marberry, The chondrocyte primary cilium, Osteoarthritis Cartilage, 22 (2014), 1071–1076.
- F. Tao, T. Jiang, H. Tao, H. Cao, and W. Xiang, Primary cilia: Versatile regulator in cartilage development, Cell Prolif, 53 (2020), e12765.
- B. Song, C. J. Haycraft, H. S. Seo, B. K. Yoder, and R. Serra, Development of the post-natal growth plate requires intraflagellar transport proteins, Dev Biol, 305 (2007), 202–216.
- N. Oliazadeh, K. F. Gorman, R. Eveleigh, G. Bourque, and A. Moreau, Identification of elongated primary cilia with impaired mechanotransduction in idiopathic scoliosis patients, Sci Rep, 7 (2017), 44260.
- C. E. de Andrea, M. Wiweger, F. Prins, J. V. Bovée, S. Romeo, and P. C. Hogendoorn, Primary cilia organization reflects polarity in the growth plate and implies loss of polarity and mosaicism in osteochondroma, Lab Invest, 90 (2010), 1091–1101.
- V. Abad, J. L. Meyers, M. Weise, R. I. Gafni, K. M. Barnes, O. Nilsson, et al., The role of the resting zone in growth plate chondrogenesis, Endocrinology, 143 (2002), 1851–1857.
- Y. Rais, A. Reich, S. Simsa-Maziel, M. Moshe, A. Idelevich, T. Kfir, et al., The growth plate’s response to load is partially mediated by mechano-sensing via the chondrocytic primary cilium, Cell Mol Life Sci, 72 (2015), 597–615.
- K. Mizuhashi, W. Ono, Y. Matsushita, N. Sakagami, A. Takahashi, T. L. Saunders, et al., Resting zone of the growth plate houses a unique class of skeletal stem cells, Nature, 563 (2018), 254–258.
- H. A. Castro-Abril, M. L. Gutiérrez, and D. A. Garzón-Alvarado, Proximal femoral growth plate mechanical behavior: Comparison between different developmental stages, Comput Biol Med, 76 (2016), 192–201.
- E. Moo, W. Herzog, S. Han, N. Abu Osman, B. Pingguan-Murphy, and S. Federico, Mechanical behaviour of in-situ chondrocytes subjected to different loading rates: a finite element study, Biomech Model Mechanobiol, 11 (2012), 983–993.
- M. E. Stender, R. A. Regueiro, and V. L. Ferguson, A poroelastic finite element model of the bone-cartilage unit to determine the effects of changes in permeability with osteoarthritis, Comput Methods Biomech Biomed Engin, 20 (2017), 319–331.
- G. Ofek, R. M. Natoli, and K. A. Athanasiou, In situ mechanical properties of the chondrocyte cytoplasm and nucleus, J Biomech, 42 (2009), 873–877.
- J. Chen, J. Irianto, S. Inamdar, P. Pravincumar, D. A. Lee, D. L. Bader, et al., Cell mechanics, structure, and function are regulated by the stiffness of the three-dimensional microenvironment, Biophys J, 103 (2012), 1188–1197.
- E. Moo, S. Han, S. Federico, S. Sibole, A. Jinha, N. Abu Osman, et al., Extracellular matrix integrity affects the mechanical behaviour of in-situ chondrocytes under compression, J Biomech, 47 (2014), 1004–1013.
- S. Rydholm, G. Zwartz, J. M. Kowalewski, P. Kamali-Zare, T. Frisk, and H. Brismar, Mechanical properties of primary cilia regulate the response to fluid flow, Am J Physiol Renal Physiol, 298 (2010), F1096–F1102.
- D. M. Allen and J. J. Mao, Heterogeneous nanostructural and nanoelastic properties of pericellular and interterritorial matrices of chondrocytes by atomic force microscopy, J Struct Biol, 145 (2004), 196–204.
- R. K. Korhonen, P. Julkunen, W. Wilson, and W. Herzog, Importance of collagen orientation and depth-dependent fixed charge densities of cartilage on mechanical behavior of chondrocytes, J Biomech Eng, 130 (2008), 021003.
- P. S. Mathieu, J. C. Bodle, and E. G. Loboa, Primary cilium mechanotransduction of tensile strain in 3D culture: Finite element analyses of strain amplification caused by tensile strain applied to a primary cilium embedded in a collagen matrix, J Biomech, 47 (2014), 2211–2217.
- Y. N. Young, M. Downs, and C. R. Jacobs, Dynamics of the primary cilium in shear flow, Biophys J, 103 (2012), 629–639.
- G. Garcia III, D. R. Raleigh, and J. F. Reiter, How the ciliary membrane is organized inside-out to communicate outside-in, Curr Biol, 28 (2018), R421–R434.
- E. R. Moore and C. R. Jacobs, The primary cilium as a strain amplifying microdomain for mechanotransduction at the cell membrane, in Molecular and Cellular Mechanobiology, S. Chien, A. J. Engler, and P. Y. Wang, eds., Physiology in Health and Disease, Springer-Verlag, New York, 2016, 3–27.
- A. Aszodi, E. B. Hunziker, C. Brakebusch, and R. Fässler, β1 integrins regulate chondrocyte rotation, G1 progression, and cytokinesis, Genes Dev, 17 (2003), 2465–2479.
- C. F. Chang, G. Ramaswamy, and R. Serra, Depletion of primary
cilia in articular chondrocytes results in reduced Gli3 repressor to activator ratio, increased Hedgehog signaling, and symptoms of early osteoarthritis, Osteoarthritis Cartilage, 20 (2012), 152–161.
- X. Yuan, R. A. Serra, and S. Yang, Function and regulation of primary cilia and intraflagellar transport proteins in the skeleton, Ann N Y Acad Sci, 1335 (2015), 78–99.
- S. R. McGlashan, C. G. Jensen, and C. A. Poole, Localization of extracellular matrix receptors on the chondrocyte primary cilium, J Histochem Cytochem, 54 (2006), 1005–1014.
- F. Barsch, T. Niedermair, A. Mamilos, V. H. Schmitt, D. Grevenstein, M. Babel, et al., Physiological and pathophysiological aspects of primary cilia—a literature review with view on functional and structural relationships in cartilage, Int J Mol Sci, 21 (2020), 4959.
- C. E. de Andrea, J. F. Zhu, H. Jin, J. V. Bovée, and K. B. Jones, Cell cycle deregulation and mosaic loss of Ext1 drive peripheral chondrosarcomagenesis in the mouse and reveal an intrinsic cilia deficiency, J Pathol, 236 (2015), 210–218.
- S. R. McGlashan, E. C. Cluett, C. G. Jensen, and C. A. Poole, Primary cilia in osteoarthritic chondrocytes: From chondrons to clusters, Dev Dyn, 237 (2008), 2013–2020.
- D. R. Rich and A. L. Clark, Chondrocyte primary cilia shorten in response to osmotic challenge and are sites for endocytosis, Osteoarthritis Cartilage, 20 (2012), 923–930.
- G. A. Ateshian, K. D. Costa, and C. T. Hung, A theoretical analysis of water transport through chondrocytes, Biomech Model Mechanobiol, 6 (2007), 91–101.
- H. Khayyeri, S. Barreto, and D. Lacroix, Primary cilia mechanics affects cell mechanosensation: A computational study, J Theor Biol, 379 (2015), 38–46.
- B. Cohen, W. M. Lai, and V. C. Mow, A transversely isotropic biphasic model for unconfined compression of growth plate and chondroepiphysis, J Biomech Eng, 120 (1998), 491–496.
- J. W. Smith, The relationship of epiphysial plates to stress in some bones of the lower limb, J Anat, 96 (1962), 58–78.
- G. De Bastiani, R. Aldegheri, L. Renzi Brivio, and G. Trivella, Chondrodiatasis-controlled symmetrical distraction of the epiphyseal plate. Limb lengthening in children, J Bone Joint Surg Br, 68 (1986), 550–556.
- P. O. Newton, Spinal growth tethering: indications and limits, Ann Transl Med, 8 (2020), 27.
- A. Molla-Herman, R. Ghossoub, T. Blisnick, A. Meunier, C. Serres, F. Silbermann, et al., The ciliary pocket: an endocytic membrane domain at the base of primary and motile cilia, J Cell Sci, 123 (2010), 1785–1795.
Copyright © 2022 P. M. Jimenez Carrion and J. L. Williams. This is an open access article distributed under the terms of the Creative Commons Attribution License, which permits unrestricted use, distribution, and reproduction in any medium, provided the original work is properly cited.