Vol. 3 (2022), Article ID 246142, 12 pages
Research Article
Fructose-Induced Cognitive Impairment Co-occurs with Morphological Changes in Dendrites and Microglia of Male Rats
Molly M. Hyer, Samya K. Dyer, Laurel V. Kovalchick, Imogen Targett, Chloe M. Burns, Alix Kloster, Amy J. Wegener, Charlie S. Sanchez, and Gretchen N. Neigh
Department of Anatomy and Neurobiology, Virginia Commonwealth University Richmond, Richmond, VA 23298, USA
Received 6 December 2021; Revised 21 March 2022; Accepted 20 May 2022; Published 3 July 2022
Molly M. Hyer, Samya K. Dyer, Laurel V. Kovalchick, Imogen Targett, Chloe M. Burns, Alix Kloster, Amy J. Wegener, Charlie S. Sanchez, and Gretchen N. Neigh, Fructose-Induced Cognitive Impairment Co-occurs with Morphological Changes in Dendrites and Microglia of Male Rats, Psychoneuroimmunology Journal, 3 (2022), art246142. doi:10.32371/pnij/246142
Fructose consumption in adolescents is accompanied by increased incidence of childhood diabetes, metabolic disorder, and obesity that can last into adulthood. Adult male rats that consumed a
high fructose diet (HFD) in adolescence have dysregulated emotional processing and upregulated gene expression of neuroinflammatory markers, yet downstream consequences on cognitive function in both sexes remain unknown. The current study aimed to determine the extent to which a HFD altered neural and microglial structure and function that may contribute to cognitive changes inmales and females. Wistar rats were fed either chow or a HFD (55% fructose) beginning on postnatal day (PND) 23 and for the duration of the study. As adults, fructose-fed males, but not females, displayed impaired cognitive flexibility on the Barnes maze task and had more reactive microglia and altered neuronal morphology throughout the dentate gyrus (DG) and CA3 regions of the hippocampus. These findings suggest that, in males, a HFD activates microglia which may contribute to functional deficits, possibly through altering dendritic structure making them disproportionally vulnerable to fructose compared to females.
fructose; cognition; sex; microglia; hippocampus
Escalating fructose consumption over recent decades has led to an increase in metabolic syndrome and diabetes [1]. Fructose driven metabolic disorders are often accompanied by changes in mental health, including anxiety and depression [2,3]. In addition to the notable decline in psychiatric function with metabolic disease, cognitive impairments can result from excessive fructose intake [4,5]. Diets high in caloric intake from sugars and fats increase risk for cognitive decline and Alzheimer’s disease [6,7,8,9], yet the mechanisms responsible remain unknown. Diet-induced cognitive decline is evident in both men and women [10,11], however, there is evidence that women may be more protected than men against diet-related changes [12]. Conversely, females can show parallel, and even worse, associated peripheral symptoms following high fructose consumption [13]. Despite these effects, little attention has been devoted to identifying sex differences in cognitive function following a fructose-rich diet and the sex-specific mechanisms driving these changes.
Diets that shift the energetic balance of the nervous system can contribute to neuroinflammation—exacerbating age-related psychomotor slowing in humans [14]. Microglia, the brain’s resident immune cells, are susceptible to diet-related activation—possibly contributing to altered neural function. Rodent models of high fructose consumption provide a powerful tool to determine the mechanisms underlying diet-induced perturbations to the brain and behavior. Fructose consumption in male rodents is accompanied by increased reactivity of microglia [15,16]. Reactive microglia can further contribute to changes in neural function by altering dendritic arborization and spine density [17]. Similarly, fructose-rich diets in males have been shown to alter these same metrics [18,19] indicating multiple points of interaction for diet and inflammation to disrupt neural structure leading to downstream consequences on behavior. While these effects have been observed in males, the potential effects of dietinduced neuroinflammation on dendritic structure have not been quantified in females, leaving a gap in our knowledge regarding the mechanisms contributing to diet-related impairments across the sexes.
Many of the observed changes in rodent behavior reflect the deficits seen in humans [10,11,20]. Male rats [21] and hamsters [4] show deficits in learning and memory tasks following consumption of a high fructose diet (HFD) due to brain insulin resistance and synaptic degradation. The fructose-induced increase in reactive microglia [15,16] observed in male rodents is accompanied by alterations in recognition and working memory performance [22]. Short-term spatial learning and memory in females appears unaltered with high fructose consumption [23] possibly due to the protective effect of estrogen [24,25], regardless of changes in synaptic respiration [26] and considerable peripheral damage [13]. Despite observed cognitive decline with elevated body mass index in women [10], the extent to which females show diet-related deficits in cognition and the mechanisms responsible remain unknown.
Here, we aimed to investigate the consequences of long-term high fructose consumption begun in adolescence in adult male and female rats. We investigated the extent to which a HFD altered spatial learning and memory performance along with microglial reactivity and dendritic complexity. As both the prefrontal cortex (PFC) and hippocampal subregions are involved in learning and memory, we analyzed microglial morphology and dendritic structure throughout the PFC, dentate gyrus (DG), CA3, and CA1. We hypothesized that males would be more vulnerable to the negative effects of fructose consumption compared to females.
Full description of animals and care procedures for Wistar rats (N = 46) were reported in a previous work by Hyer et al. [13]. Briefly, male (n = 22) and female (n = 24) Wistar rats were born in house, weaned at postnatal day (PND) 22,
and started on either a chow (Envigo Laboratory Diet 7012 Teklad LM-485) or HFD (Research Diets D050111802) consisting of 10% kcal fat and 55% kcal fructose on PND 23. Food consumption, weight, and glucose were monitored throughout the duration of the study and are reported in [13]. All animal procedures were conducted in accordance with the Institutional Animal Care and Use Committee of Virginia Commonwealth University, as well as the National Institutes of Health Guide for the Care and Use of Laboratory Animals.
On PND 80, spatial learning and memory was assessed using the Barnes maze task as previously described [27].
Briefly, after three days of habituation, acquisition learning consisted of two trials, three minutes each, to locate a goal box in the arena over five consecutive days. The arena was a circular table (121 cm diameter) with 20 equidistant holes (10 cm in diameter) around the perimeter—one of which contained a goal box, with the remaining 19 holes having false bottoms. Goal box location was counterbalanced across groups. Upon entry into the goal box, the lights were dimmed, and the hole was covered for two minutes before returning the rat to its home cage for a three-minute intertrial interval. For the reversal learning to measure cognitive flexibility, the goal box location was switched across the arena and the rats were trained for three days with two trials per day to learn the location of the new goal box. Recorded metrics include latency to locate the goal box, number of incorrect holes visited (errors), and
velocity. Data were summed or averaged across both trials. A memory probe trial was conducted after the five days of acquisition learning and after the three days of reversal learning. Rats were allowed five minutes to explore the arena. Latency to visit the location of the goal box, primary errors, and velocity were recorded.
Vaginal cytology was used to determine whether estrus cycle stage had an effect on performance in the Barnes maze task. Vaginal lavage was performed for approximately 10 days before behavioral testing to determine a pattern, then these results were used to predict cycle stage on testing days. Female Wistar rats were gently restrained and the vaginal cavity was flushed with approximately 200 uL of phosphate buffered saline (PBS). The resulting sample was smeared on a slide, then immediately imaged (while wet) at 10× magnification. Proestrus was characterized by round nucleated epithelial cells often matted in clusters, estrus was characterized by a surplus of anucleated round cells and pointed needle-like cells, metestrus produced a high concentration of neutrophils as well as anucleated round and needle-like cells, and diestrus was characterized by the presence of only neutrophils [28]. Males were gently restrained for an equivalent amount of time.
Animals were anesthetized via intraperitoneal injection of 150 mg/kg of Somnasol (Henry Schein Animal Health, Dublin, OH, USA). Brains were extracted following transcardial perfusion with saline followed by 4% paraformaldehyde. Brains were postfixed in 4% paraformaldehyde for 24 h and then stored in PBS at 4°C.
Brains were sectioned at 40 μm on the vibratome throughout the rostro-caudal extent of the PFC and hippocampus. Dried, mounted sections were rinsed in PBS and then incubated in 0.6% H2O2 in PBST for 30 min. Sections were rinsed with PBS and placed in a heated citrate buffer (10 mM citric acid, pH 6.0) for 20 min. After rinsing in PBS, tissue was blocked in 10% Normal Horse Serum (Vector, Cat. #S-2012) and 0.4% Triton x-100 (Sigma, Cat. #X100-100ML) in PBS used for the blocking buffer 90 min. Following the blocking buffer, tissue treated overnight with rabbit anti-IBA-1 antibody (1:2000,Wako Cat. #019-19741). Sections were rinsed with PBS and treated with secondary antibody goat antirabbit IgG (H+L) (1:250, Vector Laboratories, Cat. #BA-1000) in 10% Normal Goat Serum, 0.4% Triton x-100 in PBS for 1 h at room temperature. The sections were rinsed in PBS, and incubated in an ABC solution (ThermoScientific, Cat. #32020) for 30 min, rinsed, and then treated with DAB (Sigma, D4293-50SET) for 3 min. After a final rinse, sections were dried overnight, rehydrated with dH2O and immersed in Cresyl Violet. Sections were then cleared in a 70% ethanol (EtOH) solution with 6 drops of glacial acetic acid for 10 min followed by serial dehydration in 95% and 100% EtOH solution for 5 min each before final immersion in Xylene. Slides were cover slipped with Permount.
Cells were visualized on a widefield microscope (Zeiss Axio Imager, Carl Zeiss, Thornwood, NY, USA) at 100× oil immersion, and microglia morphology was assessed with Neurolucida software (MBF Biosciences, Williston, VT, USA). Ten microglia per region, five per dorsal and ventral sections, of the hippocampus were reconstructed to assess intersections with Scholl analysis rings, process length, and cell volume. Reconstructed cells were uploaded to Neurolucida Explorer and quantitative data was acquired. Microglia were sampled from the entire rostral-caudal extent of the hippocampus. As the dorsal-ventral axis of the hippocampus plays distinct roles in behavior with the dorsal region mediating learning and the ventral region being more involved in emotional regulation [29], we parsed out dorsal and ventral hippocampus to determine whether diet contributed to region-specific effects along the dorsal ventral axis. Dorsal and ventral sections were determined using Paxinos Brain Atlas [30]. Hippocampal sections prior to Figure 70 were counted as dorsal and after Figure 70 as ventral. Microglia were characterized as reactive if they displayed shorter, less complex processes evident by shorter length and simplified Sholl analysis [15,19,31,32,33]. As cell body volume is characteristic of reactive microglia following fructose diets [34], that metric was included.
A separate cohort of animals was reared identically to the cohort described in [13]. Data from these animals were reported in [26]. After 10 weeks on the chow diet or HFD, brain tissue from male and female Wistar rats (N = 24) was collected via rapid decapitation and whole hemispheres were processed for Golgi impregnation using the Rapid Golgi Staining Kit per manufacturer’s instructions (FD Neurotechnologies, Columbia, MD, USA). Hemispheres were sectioned at 100 μm on a Vibratome (Leica, Buffalo, IL, USA), mounted on slides, allowed to dry, and visualized using kit reagents per manufacturer’s instructions. Slides were cover slipped with Permount and allowed to dry prior to imaging.
Neuron morphology was analyzed via live image tracing on Neurolucida software (63× oil immersion). Neuron tracings were loaded into Neurolucida Explorer where branch points and total length were recorded. To analyze spine density, image stacks with 0.4 μm intervals were taken using a widefield light microscope at 63× oil immersion. Regions of interest included the PFC, DG dorsal region, DG ventral region, CA3 dorsal region, and CA3 ventral region. Neurolucida 360 software was used to trace and classify the spines into “filapodia,” “thin,” “mushroom,” and “stubby.” Tracings were loaded into Neurolucida Explorer software and spine quantity, classification, and density were recorded.
Data were analyzed using Graphpad Prism software (version 8.3.0; La Jolla, CA, USA). The Barnes maze data were analyzed by three-way analysis of variance (ANOVA) with the factors of sex, diet, and training day. Significant interactions were further analyzed using two-way comparisons to determine directionality of the effect. Two-way ANOVAs were used to analyze the probe task and all other neural endpoints with sex and diet as factors. Sidak’s posthoc was used to elucidate the driving variable when significant interactions were observed (P < .05).
During initial acquisition learning on the Barnes maze task, all animals successfully learned the location of the goal box over five days evidenced by a reduced latency to locate the goal box across the five training days (F(4,168) = 56.63; P < .0001; Figure 1(a)) and a reduction in number of errors committed prior to entering the goal box (F(4,210) = 35.99; P < .0001; Figure 1(b)). Sex (F(1,42) = 12.84; P = .0009; Figure 1(a)) but not diet (P > .05) altered latency to locate the goal box. There was an interaction between training day and sex (F(4,176) = 5.22; P = .0005; Figure 1(a)) such that males took longer to find the goal box on training day one compared to females (P = .002). Velocity was not altered by diet or sex (P > .05; Figure 1(c)).
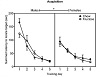
Figure 1: Males, but not females, fed the HFD displayed impaired reversal learning on the Barnes maze task. (a) Latency to locate the goal box was not altered by diet in either males or females during acquisition learning. Females had slightly faster latencies compared to males but all animals learned the task over the five training days. During acquisition learning, both (b) number of errors and (c) velocity were not altered by diet or sex. (d) Males fed the HFD had longer latencies to locate the new goal box location during reversal learning compared to chow controls. (e) Error rate was increased on training days one and two during reversal learning for the HFD males suggesting that the delayed latency to locate the new goal box was a result of incorrect hole visits. (f) Average velocity was not altered by diet. Reversal learning in females was not impacted by diet. Symbols represent mean ± SEM. ∗P < .05.
Latency to locate the new goal box (F(2,84) = 33.63; P < .0001; Figure 1(d)) and the number of errors committed prior to locating the new goal box (F(2,84) = 9.06; P = .0002; Figure 1(e)) decreased across the reversal learning days indicating that all animals learned the location of the new goal box. However, diet impacted the latency to locate the new goal box (F(1,42) = 4.13; P = .04; Figure 1(d)) and contributed to the number of errors committed prior to learning the new location (F(1,42) = 3.63; P = .06; Figure 1(e)). In males, the HFD increased the latency to learn the location of the new goal
box (F(1,20) = 7.68; P = .01; Figure 1(d)) compared to chow controls. Diet and training day interacted to alter error rate (F(2,40) = 3.61; P = .04; Figure 1(e)) showing that fructose-fed males committed more errors on the first day of reversal learning compared to chow controls (P = .004). Diet did not influence reversal learning in females (P > .05; Figures 1(d) and 1(e)). Neither diet nor sex altered velocity during reversal learning (P > .05; Figure 1(f)).
Neither diet (P > .05) nor sex (P > .05) altered the latency to locate the goal box location in either probe trial. During the second probe, diet increased the latency to visit the old goal box location learned during acquisition regardless of sex (F(1,39) = 6.48; P = .02). Neither diet (P > .05) nor sex (P > .05) influenced initial goal box selection (old or new) during the probe trial. Diet (P > .05) and sex (P > .05), likewise, did not alter the frequency of visits to the old goal box during the second probe.
Within the hippocampus, there were region-specific effects of diet and sex on microglia morphology. In the DG, diet (F(1,17) = 6.12; P = .02; Figure 2(a)) as well as an interaction between diet and sex (F(1,17) = 11.33; P = .004; Figure 2(a)) shifted the number of intersections in the Sholl analysis. Males fed the HFD had fewer intersections compared to chow controls (P = .001). Diet (F(1,17) = 5.26; P = .04; Figure 2(b)) and an interaction between diet and sex (F(1,17) = 15.01; P = .001; Figure 2(b)) modified process length in the DG. HFD males had shorter total process length compared to chow controls (P = .0007). Diet and sex did not alter cell body volume in the DG (P > .05; Figure 2(c)). Diet did not alter microglial morphology in females (P > .05; Figures 2(a), 2(b), and 2(c)).
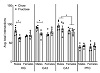
Figure 2: Males fed a HFD showed more reactive microglia in the DG and CA3 regions of the hippocampus compared to chow-fed controls. (a) Total process intersections after Sholl analysis indicated that males fed a HFD had fewer intersections
indicating less complex microglia processes compared to chow controls—indicative of a more reactive phenotype. Females showed fewer intersections than males in the CA1 region. (b) Total process length in the DG and CA3 was shorter in males fed a HFD compared to controls. Females again showed shorter process length in the CA1 region compared to males. (c) Total cell body volume was not altered by diet in either sex. No differences between any metrics were evident in the PFC. Bars represent mean ± SEM. ∗P < .05. Representative (d) ramified microglia and (e) reactive microglia. (f) Representative tracing and Sholl analysis of a microglia. Scale bar = 20 μm.
In the CA3 region, diet and sex (F(1,17) = 11.37; P = .004; Figure 2(a)) altered the number of intersections in the Sholl analysis with HFD males displaying fewer intersections compared to chow controls (P = .005). Diet (F(1,17) = 4.84; P = .04; Figure 2(b)) and an interaction between sex and diet (F(1,17) = 15.69; P = .001; Figure 2) shifted process length in the CA3. Males fed the HFD displayed less total length in area CA3 than chow controls (P = .0007). There was no effect of diet or sex on cell body volume in the CA3 (P > .05; Figure 2(c)). Females did not show altered morphology with diet in the CA3 region (P > .05; Figures 2(a), 2(b), and 2(c)).
In the CA1 region, sex altered the total number of intersections (F(1,17) = 4.64; P = .05; Figure 2(a)) and length (F(1,17) = 5.71; P = .03; Figure 2(b)) indicating that females had less complex processes than males, regardless of diet (P > .05). Sex did not modify cell body volume or nodes in area CA1 (P > .05; Figure 2(c)). There was no effect of diet on any measures of microglia morphology in the CA1 region (P > .05; Figures 2(a), 2(b), and 2(c)). There were no effects of sex (P > .05) or diet (P > .05) on microglia morphology in the PFC (Figures 2(a), 2(b), and 2(c)). Finally, total number of microglia was not altered by sex or diet in the DG, CA3, CA1 or PFC
(P > .05). There were no significant correlations between behavior in the Barnes maze task and altered metrics of microglia morphology (P > .05 for all correlations). Dorsal and ventral hippocampus did not differ in microglia morphology in any region (P > .05), so the final sample sizes were collapsed across the rostral-caudal extent of the hippocampus.
Based on the data regarding diet-induced changes in microglial morphology in the DG and CA3 regions, we investigated neuronal morphology to determine whether diet altered neurons in addition to microglia in these two regions. The PFC was used as a control region. In the DG, diet altered total dendritic length (F(1,20) = 5.36; P = .031; Figure 3(a)) and number of branch points (F(1,20) = 5.58; P = .03; Figure 3(b)) such that both males and females fed the HFD displayed shorter and less complex dendritic trees in the DG (P > .05) compared to chow-fed controls. There was no effect of sex or diet on dendritic length or branching in the CA3 or PFC (P > .05; Figures 3(a) and 3(b)). Dendritic morphology across the dorsal-ventral axis of the hippocampus did not differ in any region (P > .05) so the final sample sizes were collapsed across the rostral-caudal extent of the hippocampus.
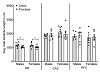
Figure 3: Consumption of a HFD reduced dendritic complexity in the DG of both males and females. (a) Dendritic length in the DG was shorter following a HFD in both males and females, but was unchanged in the CA3 and PFC. (b) Dendritic branching, in both HFD males and females, was reduced in the DG, but remained unaltered in the CA3 and PFC. Bars represent mean ± SEM. ∗P < .05. Representative images of (c) a complex dendritic tree and (d) a less complex dendritic tree. Scale bar = 20 μm.
Spine density was quantified in the dorsal and ventral regions of the DG and CA3 as well as the PFC for comparison. There were differences in spine density across the dorsal-ventral axis of the DG and CA3, so the analysis was conducted with axis as an additional variable. Spine density in the dorsal DG was not altered by diet (P > .05; Figure 4(a)) or sex (P > .05; Figure 4(a)). However, in the ventral DG, there was an interaction between sex and diet on spine density (F(1,16) = 4.67; P = .04; Figure 4(a)). Fructose-fed males had increased spine density along ventral hippocampal dendrites compared to controls (P = .05). In the CA3 region, sex and diet interacted (F(1,20) = 12.15; P = .002; Figure 4(b)) to alter spine density along ventral dendrites but not along dorsal dendrites (P > .05). In males (P = .04) and in females (P = .05), diet contributed to ventral CA3 spine density (Figure 4(b)). Males fed the HFD had increased spine density in the ventral CA3, whereas females fed the HFD had decreased spine density in this region compared to controls. In the PFC, diet (P > .05) and sex (P > .05) did not alter spine density (Figure 4(c)).
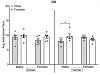
Figure 4: Spine density was altered by a HFD in the ventral portions of the DG and CA3. (a) Spine density in the dorsal DG was unaltered by diet, but was increased in males in the ventral DG. (b) Spine density in the dorsal CA3 was unaltered by diet, but was altered in the ventral region. Males fed a HFD showed increased spine density, while females fed the HFD showed reduced spine density compared to chow-fed controls. (c) Spine density in the PFC was not altered by diet. Bars represent mean ± SEM. ∗P < .05. (d) Representative images of spines along a dendritic segment with a high density of spines (top) and low density of spines (bottom). Scale bar = 10 μm.
The results reported here indicate that elevated fructose consumption contributes to changes in microglial morphology along with reduced complexity of dendritic structure in brain regions involved in learning and memory in male rats. These changes in neural morphology are accompanied by impaired cognitive flexibility. Despite the diet-induced consequences observed here in males, females do not show microglial reactivity and impaired cognition despite a moderate decrease in spine density and dendritic complexity in the hippocampus. While the animals in the current study were fed a HFD for 10 weeks, they did not display altered weight gain or shifted caloric efficiency, suggesting that even in the absence of these more traditional markers of metabolic syndrome, fructose can contribute to neural dysfunction and neuroinflammation in males.
Microglia are the innate immune cells of the brain and are highly responsive to the neural environment. Activated microglia can directly contribute to altered neural function by degrading synapses and altering neuronal firing [17,35]. Here, male rats that consumed a HFD from adolescence through adulthood displayed more reactive microglia in the DG and CA3 subregions of the hippocampus, while the CA1 and PFC remained similar to controls. The hippocampal subregions comprise the trisynaptic pathway that is responsible for learning and shorter-term memory with the PFC contributing to long-term memory [36]. The DG, in particular, is a highly vascularized region with its proximity to the ventricles, making it susceptible to external input such as diet-related nutrients. Granular cells in the DG synapse with the mossy fibers of the CA3 for a complex interconnectivity that is essential to learning and memory formation [36]. Here, males fed a HFD showed reactive microglia in the DG and CA3 regions along with a reduced complexity of neurons in the DG. It is possible that fructose consumption activated microglia in these vulnerable regions and that the reactive microglia contributed to dendritic pruning. Reactive microglia activate the complement cascade which directly contributes to changes in dendritic structure [17,35]. Male rats fed a HFD show increased gene expression for a number of complement proteins in the hippocampus [37]. It is possible that the diet-induced reactivity drives microglia to alter the complement signaling pathways contributing to changes in dendritic structure and downstream shifts in behavior.
Previous work has shown diet-induced consequences on glial and neuronal structure in males. Just seven days of consumption of a high fat, HFD did not alter microglia in the CA1 and DG, however, there was an increase in astrocytic reactivity and reduced dendritic complexity in these two regions with the experimental diet [19]. It is possible that astrocytes, which maintain end feet along the neural vasculature [38], are more susceptible to the immediate consumption of fructose whereas microglia respond only after an extended period. Eight months of a high fat, glucose, and fructose diet led to decreased spine density along both apical and basal segments of the CA1 pyramidal neurons in male rats as well as impaired long-term potentiation, while dendritic length and complexity remained unchanged. This compromised neural signaling and structure was accompanied by impaired cognition [18]. Taken together, these data suggest that both diet content and duration can determine the pattern of hippocampal changes. Shorter-term diets may compromise more vulnerable targets while longer-term consumption migrates throughout the pathways involved in learning and memory.
While males showed considerable neuroinflammation and compromised dendritic architecture, parallel effects in females were minimal. Females fed the HFD showed compromised dendritic structure in the DG and reduced spine density in the ventral CA3 region. Microglia reactivity was not altered in females fed the fructose-enriched diet. Previous work has shown that effects of fructose are blunted in females compared to males [12], and sex hormones may mediate fructose metabolism as females show far lower sensitivity to insulin compared to males [39,40], yet this difference dissolves following menopause [41]. While this protection is considerable, extended consumption of elevated fructose does put females at risk for peripheral damage such as non-alcoholic fatty liver disease [13]. Furthermore, female rats that consume high fructose beginning in adolescence show increased depressive-like behavior as adults along with altered synaptic mitochondrial respiration [26]. The ventral hippocampus contributes to emotional learning and memory [29]. As the current work observed decreased spine density in the ventral apical CA3 region, it is possible that longer-term consumption in females can lead to neural impairments and emotional dysregulation mediated by damage to the ventral hippocampus. It remains unknown if a longer continuation of a HFD would induce neuroinflammation and/or cognitive impairments in females.
Male rats that consumed elevated fructose for ten weeks beginning in adolescence displayed impaired reversal learning on a spatial task as adults. Females did not show any diet-related changes in cognitive performance. Similar to the changes observed to brain structure, it is likely that diet-induced changes in cognitive function are dependent on the type and duration of the diet. Male mice fed a high fat diet for eleven weeks beginning in adolescence showed impaired memory and spatial learning. Like the current work, the extended high fat diet compromised cognitive flexibility. Importantly, when this
diet was begun in adulthood, there were no evident changes to cognitive performance [42,43]. Likewise, previous work from our group has demonstrated that the negative consequences of high fructose consumption are dependent on adolescent consumption compared to beginning the diet in adulthood [44]. Impairments in the water maze were evident in initial learning performance in male rats fed a high fat, glucose, and fructose diet for eight months. However, path length was also longer, suggesting that mobility may have contributed to the observed deficit [18]. These data highlight the various aspects of cognitive function that can be impaired by diet and emphasize that the specific consequences are nuanced based on the type of caloric intake and the duration of consumption. Finally, females, while underrepresented in the literature, appear to not display diet-related impairments following fructose consumption. Whether or not cognitive decline occurs following a high-fat diet or a blended, high caloric diet remains to be seen.
There is considerable interest in the permanence of these diet-induced effects. When male mice were put on a standard control diet for three months in adulthood following adolescent long-term consumption of a high fat diet, observed cognitive decline was reversed [43]. Similar effects are observed in the periphery such as reductions in obesity, metabolic syndrome, and non-alcoholic fatty liver disease [45]. It is likely that caloric intake manipulates energy expenditure and that, overtime, the body and brain attempt to compensate for the altered energy input. In overweight men and women, high fructose intake for 10 weeks leads to a decrease in resting energy expenditure which contributes to a build-up of excess energy substrates [46]. As the hypothalamus is the primary regulator of homeostasis, diet-induced perturbations may influence neural function through energetic imbalance. Indeed, male rats fed a HFD from adolescence into adulthood displayed a global reduction in synaptosomal oxygen consumption rate throughout the brain while females consuming high fructose showed the opposite effect [26]. Furthermore, there is considerable evidence that exercise alleviates many of the negative consequences of fructose consumption such as cardiovascular autonomic dysfunction [47] and a variety of metabolic syndrome symptoms [48]. Given these data, it is possible that the cognitive decline observed following extended consumption of high caloric diets may be a result of altered neurometabolism that contributes to impaired neural structure and function as well as neuroinflammation. By shifting away from a compromised diet, energy utilization in the brain can re-establish a baseline leading to improved neural function.
It should be noted that, while there were no differences between the chow-fed and fructose-fed females, both groups were similar to the fructose-fed males in cognitive performance and microglia reactivity. This suggests that females, at baseline, score lower on tests of cognitive flexibility and have increased microglia reactivity compared to males. Thus, we may be observing a floor effect whereby fructose consumption cannot worsen cognitive flexibility or microglia reactivity in females. Previous work suggests that females do, in fact, have worse cognitive performance in some spatial tasks, such as the Barnes maze, compared to males. However, other metrics of cognitive flexibility that do not depend on spatial performance show that females outperform males (reviewed in [49]). Thus, it is not unexpected that we would observe this effect here. Microglial reactivity at baseline varies between males and females across different brain regions throughout development. In the hippocampus, adult females show more reactive microglia compared to males [50]. Taken together, the previous literature supports lower spatial performance and increased hippocampal microglial reactivity in females compared to males, as we observed here. It remains unlikely that the lack of a fructose effect in these domains is due to a floor effect as both current Barnes maze performance and microglial reactivity have been shown to be reduced in females following disruptions such as ovariectomy [27] or stress [51]. It appears most likely that consumption of 55% fructose for 12 weeks is not sufficient to worsen spatial learning and increase microglia reactivity in female rats as compared to male rats.
These data highlight the nuanced consequences of diet type and duration and emphasize the importance of sex as a biological variable. The data presented here, combined with the existing literature, indicate that the brain is susceptible to diet-related decline. The scope of the decline appears specific to the type of diet and the extent of consumption. While incidence of diabetes and obesity are directly related to excessive caloric intake [52], here, we observed no changes in caloric efficiency. This suggests that although excess caloric intake can induce severe metabolic consequences, the source of calories may be detrimental, even without metabolic symptoms. The demonstration here of considerable deficits in males on a high fructose diet without any changes in caloric efficiency, weight gain or glucose indicates a vulnerability of the brain to energy intake that may not be reflected by peripheral metrics. The absence of negative neural outcomes in females exposed to high fructose, despite previous observed peripheral decline [13], suggests a powerful role for sex hormones in buffering the cerebral impact of diet. Here, the reported observation of compromised neural function in the absence of observed peripheral disease suggests that the inability of patients suffering from diet-related diseases to change their lifestyle habits may be a result of cognitive impairment [45,53,54]. Future work to understand how diet and energy intake influence neurometabolism and neural structure across the sexes will be an important advancement in understanding diet-related disease.
This work was supported by the Department of Anatomy and Neurobiology at Virginia Commonwealth University. MMH was supported by the National Institute of General Medical Sciences
(K12GM093857). Microscopy was performed at the VCU Microscopy Facility, supported, in part, by funding from NIH-NCI Cancer Center Support Grant (P30 CA016059).
The authors declare that they have no conflict of interest.
- A. C. Rutledge and K. Adeli, Fructose and the metabolic syndrome: pathophysiology and molecular mechanisms, Nutr Rev, 65 (2007), S13–S23.
- G. Zhao, E. S. Ford, S. Dhingra, C. Li, T. W. Strine, and A. H. Mokdad, Depression and anxiety among US adults: associations with body mass index, Int J Obes (Lond), 33 (2009), 257–266.
- K. G. Kahl, U. Schweiger, C. Correll, C. Müller, M. L. Busch, M. Bauer, et al., Depression, anxiety disorders, and metabolic syndrome in a population at risk for type 2 diabetes mellitus, Brain Behav, 5 (2015), e00306.
- R. Agrawal and F. Gomez-Pinilla, ‘Metabolic syndrome’ in the brain: deficiency in omega-3 fatty acid exacerbates dysfunctions in insulin receptor signalling and cognition, J Physiol, 590 (2012), 2485–2499.
- J. E. Beilharz, J. Maniam, and M. J. Morris, Diet-induced cognitive deficits: the role of fat and sugar, potential mechanisms and nutritional interventions, Nutrients, 7 (2015), 6719–6738.
- J. A. Luchsinger, M. X. Tang, S. Shea, and R. Mayeux, Caloric intake and the risk of Alzheimer disease, Arch Neurol, 59 (2002), 1258–1263.
- A. Lloret, P. Monllor, D. Esteve, A. Cervera-Ferri, and M. A. Lloret, Obesity as a risk factor for Alzheimer’s disease: Implication of leptin and glutamate, Front Neurosci, 13 (2019), 508.
- F. Akhter, D. Chen, A. Akhter, A. A. Sosunov, A. Chen, G. M. McKhann, et al., High dietary advanced glycation end products impair mitochondrial and cognitive function, J Alzheimers Dis, 2020 (76), 165–178.
- L. M. P. Wesselman, D. M. van Lent, A. Schröder, O. van de Rest, O. Peters, F. Menne, et al., Dietary patterns are related to cognitive functioning in elderly enriched with individuals at increased risk for Alzheimer’s disease, Eur J Nutr, 60 (2021), 849–860.
- M. Cournot, J. C. Marquié, D. Ansiau, C. Martinaud, H. Fonds, J. Ferrières, et al., Relation between body mass index and cognitive function in healthy middle-aged men and women, Neurology, 67 (2006), 1208–1214.
- A. Dahl, L. B. Hassing, E. Fransson, S. Berg, M. Gatz, C. A. Reynolds, et al., Being overweight in midlife is associated with lower cognitive ability and steeper cognitive decline in late life, J Gerontol A Biol Sci Med Sci, 65 (2010), 57–62.
- C. Couchepin, K. A. Lê, M. Bortolotti, J. A. da Encarnaçao, J. B. Oboni, C. Tran, et al., Markedly blunted metabolic effects of fructose in healthy young female subjects compared with male subjects, Diabetes Care, 31 (2008), 1254–1256.
- M. M. Hyer, S. K. Dyer, A. Kloster, A. Adrees, T. Taetzsch, J. Feaster, et al., Sex modifies the consequences of extended fructose consumption on liver health, motor function, and physiological damage in rats, Am J Physiol Regul Integr Comp Physiol, 317 (2019), R903–R911.
- L. J. T. Balter, S. Higgs, S. Aldred, J. A. Bosch, and J. E. Raymond, Inflammation mediates body weight and ageing effects on psychomotor slowing, Sci Rep, 9 (2019), 15727.
- A. Granholm, H. Bimonte-Nelson, A. Moore, M. Nelson, L. Freeman, and K. Sambamurti, Effects of a saturated fat and high cholesterol diet on memory and hippocampal morphology in the middle-aged rat, J Alzheimers Dis, 14 (2008), 133–145.
- L. Freeman, V. Haley-Zitlin, C. Stevens, and A. Granholm, Diet-induced effects on neuronal and glial elements in the middle-aged rat hippocampus, Nutr Neurosci, 14 (2011), 32–44.
- B. Stevens, N. J. Allen, L. E. Vazquez, G. R. Howell, K. S. Christopherson, N. Nouri, et al., The classical complement cascade mediates CNS synapse elimination, Cell, 131 (2007), 1164–1178.
- A. M. Stranahan, E. D. Norman, K. Lee, R. G. Cutler, R. S. Telljohann, J. M. Egan, et al., Diet-induced insulin resistance impairs hippocampal synaptic plasticity and cognition in middle-aged rats, Hippocampus, 18 (2008), 1085–1088.
- E. Calvo-Ochoa, K. Hernández-Ortega, P. Ferrera, S. Morimoto, and C. Arias, Short-term high-fat-and-fructose feeding produces insulin signaling alterations accompanied by neurite and synaptic reduction and astroglial activation in the rat hippocampus, J Cereb Blood Flow Metab, 34 (2014), 1001–1008.
- I. Aeberli, M. Hochuli, P. A. Gerber, L. Sze, S. B. Murer, L. Tappy, et al., Moderate amounts of fructose consumption impair insulin sensitivity in healthy young men: a randomized controlled trial, Diabetes Care, 36 (2013), 150–156.
- A. P. Ross, T. J. Bartness, J. G. Mielke, and M. B. Parent, A high fructose diet impairs spatial memory in male rats, Neurobiol Learn Mem, 92 (2009), 410–416.
- M. Yu, H. Huang, S. Dong, H. Sha, W. Wei, and C. Liu, High mobility group box-1 mediates hippocampal inflammation and contributes to cognitive deficits in high-fat high-fructose diet-induced obese rats, Brain Behav Immun, 167–177 (2019), 82.
- E. C. Bruggeman, C. Li, A. P. Ross, J. M. Doherty, B. F. Williams, K. J. Frantz, et al., A high fructose diet does not affect amphetamine self-administration or spatial water maze learning and memory in female rats, Pharmacol Biochem Behav, 99 (2011), 356–364.
- H. Vasudevan, H. Xiang, and J. H. McNeill, Differential regulation of insulin resistance and hypertension by sex hormones in fructose-fed male rats, Am J Physiol Heart Circ Physiol, 289 (2005), H1335–H1342.
- N. Vasudevan and D. W. Pfaff, Non-genomic actions of estrogens and their interaction with genomic actions in the brain, Front Neuroendocrinol, 29 (2008), 238–257.
- A. Kloster, M. M. Hyer, S. Dyer, C. Salome-Sanchez, and G. N. Neigh, High fructose diet induces sex-specific modifications in synaptic respiration and affective-like behaviors in rats, Neuroscience, 454 (2021), 40–50.
- M. M. Hyer, G. A. Shaw, P. Goswamee, S. K. Dyer, C. M. Burns, E. Soriano, et al., Chronic adolescent stress causes sustained impairment of cognitive flexibility and hippocampal synaptic strength in female rats, Neurobiol Stress, 14 (2021), 100303.
- M. C. Cora, L. Kooistra, and G. Travlos, Vaginal cytology of the
laboratory rat and mouse: review and criteria for the staging of the estrous cycle using stained vaginal smears, Toxicol Pathol, 43 (2015), 776–793.
- M. A. Kheirbek, L. J. Drew, N. S. Burghardt, D. O. Costantini, L. Tannenholz, S. E. Ahmari, et al., Differential control of learning and anxiety along the dorsoventral axis of the dentate gyrus, Neuron, 77 (2013), 955–968.
- K. Franklin and G. Paxinos, The Mouse Brain in Stereotaxic Coordinates, Academic Press, New York, 3rd ed., 2007.
- K. Gzielo, M. Kielbinski, J. Ploszaj, K. Janeczko, S. P. Gazdzinski, and Z. Setkowicz, Long-term consumption of high-fat diet in rats: effects on microglial and astrocytic morphology and neuronal nitric oxide synthase expression, Cell Mol Neurobiol, 37 (2017), 783–789.
- N. G. Deshpande, J. Saxena, T. G. Pesaresi, C. D. Carrell, G. B. Ashby, M. K. Liao, et al., High fat diet alters gut microbiota but not spatial working memory in early middle-aged Sprague Dawley rats, PLoS One, 14 (2019), e0217553.
- C. Y. Ho, Y. T. Lin, H. H. Chen, W. Y. Ho, G. C. Sun, M. Hsiao, et al., CX3CR1-microglia mediates neuroinflammation and blood pressure regulation in the nucleus tractus solitarii of fructose-induced hypertensive rats, J Neuroinflammation, 17 (2020), 185.
- W. C. Liu, C. W. Wu, M. H. Fu, Y. L. Tain, C. K. Liang, C. Y. Hung, et al., Maternal high fructose-induced hippocampal neuroinflammation in the adult female offspring via PPARγ-NF-κB signaling, J Nutr Biochem, 81 (2020), 108378.
- D. P. Schafer, E. K. Lehrman, A. G. Kautzman, R. Koyama, A. R. Mardinly, R. Yamasaki, et al., Microglia sculpt postnatal neural circuits in an activity and complement-dependent manner, Neuron, 74 (2012), 691–705.
- C. E. Ribak and L. A. Shapiro, Ultrastructure and synaptic connectivity of cell types in the adult rat dentate gyrus, Prog Brain Res, 163 (2007), 155–166.
- C. S. Harrell, C. Zainaldin, D. McFarlane, M. M. Hyer, D. Stein, I. Sayeed, et al., High-fructose diet during adolescent development increases neuroinflammation and depressive-like behavior without exacerbating outcomes after stroke, Brain Behav Immun, 73 (2018), 340–351.
- J. I. Alvarez, T. Katayama, and A. Prat, Glial influence on the blood brain barrier, Glia, 61 (2013), 1939–1958.
- D. Galipeau, S. Verma, and J. McNeill, Female rats are protected against fructose-induced changes in metabolism and blood pressure, Am J Physiol Heart Circ Physiol, 283 (2002), H2478–H2484.
- D. Galipeau, L. Yao, and J. McNeill, Relationship among hyperinsulinemia,
insulin resistance, and hypertension is dependent on sex, Am J Physiol Heart Circ Physiol, 283 (2002), H562–H567.
- I. Macdonald, Influence of fructose and glucose on serum lipid levels in men and pre- and postmenopausal women, Am J Clin Nutr, 18 (1966), 369–372.
- C. Boitard, N. Etchamendy, J. Sauvant, A. Aubert, S. Tronel, A. Marighetto, et al., Juvenile, but not adult exposure to high-fat diet impairs relational memory and hippocampal neurogenesis in mice, Hippocampus, 22 (2012), 2095–2100.
- C. Boitard, S. L. Parkes, A. Cavaroc, F. Tantot, N. Castanon, S. Layé, et al., Switching adolescent high-fat diet to adult control diet restores neurocognitive alterations, Front Behav Neurosci, 10 (2016), 225.
- C. S. Harrell, J. Burgado, S. D. Kelly, Z. P. Johnson, and G. N. Neigh, High-fructose diet during periadolescent development increases depressive-like behavior and remodels the hypothalamic transcriptome in male rats, Psychoneuroendocrinology, 62 (2015), 252–264.
- N. Alhalel, S. M. Schueller, and M. J. O’Brien, Association of changes in mental health with weight loss during intensive lifestyle intervention: does the timing matter?, Obes Sci Pract, 4 (2018), 153–158.
- C. L. Cox, K. L. Stanhope, J. M. Schwarz, J. L. Graham, B. Hatcher, S. C. Griffen, et al., Consumption of fructose-sweetened beverages for 10 weeks reduces net fat oxidation and energy expenditure in overweight/obese men and women, Eur J Clin Nutr, 66 (2012), 201–208.
- D. da Silva Dias, N. Bernardes, F. F. Stoyell-Conti, C. P. dos Santos, A. A. de Araujo, S. Llesuy, et al., Impact of combined exercise training on the development of cardiometabolic and neuroimmune complications induced by fructose consumption in hypertensive rats, PLoS One, 15 (2020), e0233785.
- F. Er, E. Zorba, M. Günay, M. Koz, C. Yılmaz, O. T. Paşaoğlu, et al., Effect of exercise and quercetin in rats with metabolic syndrome induced with fructose, Metab Syndr Relat Disord, 20 (2022), 57–66.
- S. Yagi and L. A. M. Galea, Sex differences in hippocampal cognition
and neurogenesis, Neuropsychopharmacology, 44 (2019), 200–213.
- J. M. Schwarz, P. W. Sholar, and S. D. Bilbo, Sex differences in microglial colonization of the developing rat brain, J Neurochem, 120 (2012), 948–963.
- M. Bekhbat, D. Mukhara, M. G. Dozmorov, J. C. Stansfield, S. D. Benusa, M. M. Hyer, et al., Adolescent stress sensitizes the adult neuroimmune transcriptome and leads to sex-specific microglial and behavioral phenotypes, Neuropsychopharmacology, 46 (2021), 949–958.
- L. S. Gross, L. Li, E. S. Ford, and S. Liu, Increased consumption of refined carbohydrates and the epidemic of type 2 diabetes in the United States: an ecologic assessment, Am J Clin Nutr, 79 (2004), 774–779.
- R. R. Rubin, T. A. Wadden, J. L. Bahnson, G. L. Blackburn, F. L. Brancati, G. A. Bray, et al., Impact of intensive lifestyle intervention on depression and health-related quality of life in type 2 diabetes: the Look AHEAD trial, Diabetes Care, 37 (2014), 1544–1553.
- M. Hersey, J. L. Woodruff, N. Maxwell, A. T. Sadek, M. K. Bykalo, I. Bain, et al., High-fat diet induces neuroinflammation and reduces the serotonergic response to escitalopram in the hippocampus of obese rats, Brain Behav Immun, 96 (2021), 63–72.
Copyright © 2022 Molly M. Hyer et al. This is an open access article distributed under the terms of the Creative Commons Attribution License, which permits unrestricted use, distribution, and reproduction in any medium, provided the original work is properly cited.