Vol. 2 (2019), Article ID 236073, 6 pages
Research Article
New Manganese Complexes Showing Axially-Compressed Octahedral Geometry: Structure and Magnetic Properties
Samir Mameri
Léonardo Building, Office Léo-015, IUT Robert Schuman, 72 route du Rhin, 67411 Illkirch, France
Received 6 March 2019; Revised 21 May 2019; Accepted 1 July 2019; Published 31 July 2019
Samir Mameri, New Manganese Complexes Showing Axially-Compressed Octahedral Geometry: Structure and Magnetic Properties, Journal of Transition Metal Complexes, 2 (2019), art236073. doi:10.32371/jtmc/236073
The straightforward combination of commercial picolinaldehyde and 2-aminopyridine led to the preparation of N,1-di(pyridin-2-yl)methanimine ligand HL1. Under aerobic conditions, HL1 self-assembles with manganese (Mn) ions to afford two high-spin mononuclear Mn(III) complexes of general formula [MnL22]+Cl (1) and [MnL22]+(NO3) (2). Their synthesis is mediated via (in situ) ligand transformation and oxidation of initial Mn(II) ions. Such unexpected reorganization contributes in the stabilization of the complexes. The mononuclear compounds 1 and 2 display unusual Jahn-Teller distortion along the z-axis, as evidenced by single-crystal X-ray diffraction and do exhibit an S = 2 ground spin state.
Unexpected metal-based reorganization of parent ligand has led to the preparation of two new Mn(III)-based mononuclear complexes showing Jahn-Teller distortion, resulting in an axially-compressed octahedral geometry.
self-assembly; Jahn-Teller distortion; manganese(III); crystal structure; magnetic properties
Attempts to synthesize mimics of manganese(III) (Mn(III)) active centers result from the appreciation of the crucial role played by Mn in vital biochemical processes, notably the photosynthetic oxidation of water [1,2] in chloroplasts and the catalytic decomposition of superoxide by the bacterial enzyme superoxide dismutase [3,4]. Thus, a variety of high-valent Mn-based systems exhibiting attractive structural, magnetic, and spectroscopic features have been reported (see [5] and references therein). But, the mechanism by which this metal performs its biochemical activities is still the subject of intense investigation [6,7].
Small molecules provide detailed chemical studies that can provide chemical precedence for reactions postulated to occur in biological systems. Once known, these reactions can be experimentally probed in greater detail via systematic variation of both the metal complexes and their substrates; a level of detail difficult to achieve in biological systems. Lipoxygenases (LOs) are mononuclear non-heme metalloenzymes that are seemingly ubiquitous
in aerobic organisms, which have been found in a wide variety of plants, animals, and fungi [8,9,10]. A recently characterized lipoxygenase from the fungus Gaeumannomyces graminis was proved to contain a single Mn site that is hypothesized to oxidize substrates
in a manner similar to iron-lipoxygenases (Fe-LOs). The proclivity of Mn(III) to undergo a Jahn-Teller distortion
is well known. The Jahn-Teller effect is most often encountered in octahedral complexes of the transition metals. Such complexes distort along one of the molecular fourfold axes (always labeled the z-axis), which has the effect of both removing the orbital and electronic degeneracies and lowering the overall energy. The distortion normally takes the form of elongating the bonds to the ligands lying along the z-axis, but occasionally occurs as a shortening of these bonds instead.
The emergence of high-spin Mn(III) pseudo-octahedral complexes compressed along the
z-axis is quite recent (see [11] and references therein). In octahedral complexes, the Jahn-Teller effect is most pronounced when an odd number
of electrons occupy the eg orbitals (i.e., in d9, low-spin
d7 or high-spin d4 complexes, all of which have doubly-degenerate ground states). The same is true in tetrahedral complexes; distortion is less because there is less stabilization to be gained because the ligands are not pointing directly at the orbitals. The literature is replete with examples of high-spin Mn(III) complexes illustrating a tetragonal elongation and a rhombic distortion [12]. However, complexes of this metal ion that display
axially compressed octahedral environments are extremely few and far between: only a handful has been reported to date [13,14,15]. These latter are interesting by nature, all the more ever since the activity in a biological system was proved to be linked to a compressed rather than an elongated octahedron [16]. It is thus necessary to identify the factors that favor a tetragonally compressed geometry in these Mn(III) compounds. It is this commitment with which an inorganic chemist shall be concerned.
The paucity of Jahn-Teller compressed complexes is probably due to the lack of synthetic endeavor in this direction. The interest in trivalent Mn also arises from the possibility that at least one of the Mn atoms in the oxygen-evolving complex in photosystem II9 of green plants is in this oxidation state. As part of our contribution to the development of the co-ordination chemistry of Mn(III), we have synthesized the mononuclear complexes 1 and 2 and characterized them by single-crystal X-ray crystallography.
The mixed-donor N,1-di(pyridin-2-yl)methanimine ligand (HL1), subtly combining Schiff-base and tripodal alkoxy units, was chosen primarily to probe the effect of the nature of polydentate ligands on the type of
Jahn-Teller distortion to be adopted by the resulting Mn(III) complexes (see Figure 1). Brodie and co-workers first provide structural evidence of an axially compressed,
rhombically distorted, octahedral geometry of a mononuclear Mn(III) complex containing two tridentate ligands [17]. They have treated this distortion as a pseudo-Jahn-Teller compression because of the heteroleptic nature of the ligand. Only in a mononuclear Mn(III) complex with identical monodentate ligands can we expect to observe unambiguously the full extent of a Jahn-Teller distortion.
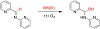
Figure 1: Ligand structure-reorganization mediated by a Mn(III) ion.
For decades, interest in coordination chemistry has centered on transition-metal complexes with Schiff-base ligands. Nevertheless, it is important to note that the axially compressed octahedral Mn(III) complexes known so far have been built-up with stereochemically flexible non-Schiff-base ligands. We report herein the synthesis, structural characterization and magnetic properties of
two new mononuclear Mn(III) complexes with a predesigned Schiff-base tripodal ligand. X-ray single-crystal analyses revealed that both compounds do exhibit compressed Jahn-Teller axes and thus represent ideal examples to ascertain the factors which govern the type of octahedral distortion to be adopted by a given high-spin d4 system.
Ligand HL1 was obtained—as a pale crystalline solid in quantitative yield via a one-pot reaction—by mixing in an acetonitrile solution the corresponding aldehyde and amine (1 equiv.) in aerobic conditions at
room temperature (RT) and further washings with cold acetonitrile and EtOH (to remove excess of amine). Compounds 1 and 2 have been both synthesized in aerobic conditions at RT by mixing a base-free acetonitrile
solution containing equimolar amounts of ligand HL1 and the corresponding chloride or nitrate Mn(II) salts, respectively. X-ray quality crystals were obtained after slow evaporation within a few days. Various reaction conditions such as reaction components, stoichiometry, concentration, reaction time, pH, and temperature were investigated. We report here the optimum conditions that we have established.
The two systems are unique in that they are (i) Schiff-base/tripodal alkoxy combining-ligands [18], (ii) Schiff/non-Schiff base-based coordinating ligands; and (iii) α-hydroxyled Schiff-base active ligands (the latter being unexpected as the hydrolysis
of a Schiff-base leads to the recovery of the corresponding aldehyde and amine). The present Mn(III) ion complexation can
be thus potentially regarded as a C−H activation pathway [19]. The present syntheses can be seen as in situ ligand transformation + oxidation of starting Mn(II) ions (see Scheme 1). Virtually, all high-spin Mn(III) complexes with polydentate Schiff-base ligands undergo a Jahn-Teller distortion as a means to lift the double degeneracy of the eg electron at the metal center. The present systems include non-Schiff-base complexing ligands, which supports their attractiveness.
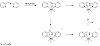
Scheme 1: Proposed mechanism for the metal-mediated ligand transformation.
To the crystallographic structure, the HL1-to-H2L2 transformation could notably be easily appreciated using IR spectroscopy, with the disparition of the imine bond being accompanied with the apparition of the C−O, C−N, and
N−H elongated bonds at ca. 1,050 cm−1, 1,350 cm−1, and 3,350 cm−1, respectively.
Compounds 1 and 2 crystallize in the monoclinic system space group P21/n or Cc, respectively. Their molecular structure are very similar and
consist of mononuclear species which are coordinated by two pyridine nitrogen and
one in situ-generated hydroxylate oxygen atoms. The structure of
complex 1 is depicted in Figure 2. Thermal ellipsoid plots and selected bond distances of complexes 1 and 2 are shown in Figure 3 and Table 1, respectively.
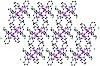
Figure 2: Crystal Structure of [MnL22]3+ along the b axes. Color code: C, grey; N, blue; O, red, Mn, purple; Cl, green.
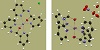
Figure 3: Thermal ellipsoid plots of complexes 1 (left) and 2 (right), set at a 50% probability level. Identification of the atoms: Mn (purple), O (red), N (blue), H (white), C (dark-grey).
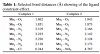
Table 1: Selected bond distances (Å) showing of the ligand constraint-effect.
Compound 1 crystallizes in the monoclinic space group P21/n with a = 12.8565(12) Å, b = 10.7374(6) Å, c = 15.7085(16) Å,
β = 95.62(1), and V = 2158.07(173) Å3, while complex 2 does crystallize in the monoclinic space group Cc with a = 11.7460(7) Å, b = 14.1705(9) Å, c = 14.9108(9) Å, β = 102.97(0), and V = 2418.51(61) Å3.
Each of these cations possesses a crystallographic imposed center of symmetry at the metal center. The mean Mn(III)-Ohydroxylate distance (1.8435–1.8732 Å) compares favorably with the reported Mn(III)-Onaphtolate distance in the aforementioned published system (1.8841–1.8852 Å), and is significantly shorter than the mean Mn(III)-Ocarboxylate and Mn(III)-Namine distances of 2.0637 Å and 2.2082 Å, respectively, in the co-ordination sphere of [MnL22]3+. These latter two distances are intermediate between those of normal and Jahn-Teller elongated bonds. Mn(III)-Ohydroxylate bonds have been reported to be normal in the 2.180–2.370 Å range. Normal Mn(III)-Namine bonds have been quoted in the 1.954–2.114 Å range, whereas those
that are Jahn-Teller elongated have been observed in the 2.341–2.414 Å range. The geometry at the metal center in the structure of
[MnL22]3+ can be truly regarded as compressed, rhombically distorted, octahedral, imposed by a combination of Jahn-Teller distortion and
ligand constraints. Due to the Janh-Teller effect, the coordination environment of Mn(III) is always distorted, these tridentate ligands usually induce a compressed distortion in the oxo-bridge direction with the distance ranging from
1.843 Å to 1.873 Å. In the cell, the mononuclear units form one-dimensional chains (see Figure 2).
Because of the differences in the donor abilities of the hydroxo oxygen and the amine nitrogens, the mixed-donor ligand environment is expected to contribute to the rhombic component of the structure of the complex. The nature of the framework of the polydentate ligand influences the extent to which the Jahn-Teller distortion will be manifested as the ligand imposes its preferred bite angles. Complex [MnL22]+ exemplifies the conflict between stabilization from the Jahn-Teller effect and the stereochemical requirements of the chelate ring system.
Analyzing the crystal structures of complexes 1 and 2, we have found short CH/π and π/π stacking interactions. Such interactions, although weak, can mediate communication between Mn(III) ions. Therefore, we did magnetic measurements analyzing these compounds as binuclear Mn(III) compounds. The χT products at RT for complexes 1 and 2 are 6.5 cm3Kmol-1 and
6.7 cm3Kmol-1, respectively (see Figure 4). These values are in good agreement with the expected value (6.0 cm3Kmol-1) for two spin-only Mn(III) ions (S = 2, g = 2, C = 3.0 cm3Kmol-1). When the temperature is lowered, the χT product stays almost constant from 300 K till 30 K and then decreases steadily to
reach 3.8 cm3Kmol-1 and 3.2 cm3Kmol-1, respectively, at 1.8 K.
This behavior is typically observed when antiferromagnetic interactions between magnetic
centers are dominant. As seen from the crystal structures, the closest Mn(III) ions are spaced at a distance of ∼6.14 Å. The interaction between them is mostly mediated by CH/π and π/π stacking interactions. Therefore, the magnetic interactions between the Mn(III) ions are likely to be very weak.
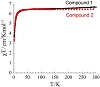
Figure 4: Plots of χT versus T at the applied field of 0.1 T for compounds 1 and 2.
The field dependences of magnetization measurements at low temperatures are very similar (see Figure 5) and reveal both a continuous increase of
the magnetization and the absence of a saturation of the magnetization (that reaches
ca. 7.4 μB at 7 T). This behavior suggests the presence of low-lying excited states that might be populated when a field is applied (i.e.,
the antiferromagnetic interactions are weak in this complex and are
easily overcome by the applied field). Indeed at high field, the interactions between the weak antiferromagnetically coupled Mn(III) ions should be completely overcome and then one would expect a saturation of the magnetization at 8 μB for all the spins parallel.
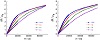
Figure 5: Plots of magnetization (M) versus applied magnetic field (H) for complex 1 (left) and complex 2 (right) at the indicated temperatures.
Manipulations were performed under aerobic conditions. Commercial reagents and solvents were used as purchased (Aldrich). FTIR spectra were recorded with a Nicolet 380 apparatus. Elemental analyses were obtained at the analytical facility
of the IUT Robert Schuman, University of Strasbourg. The magnetic susceptibility measurements were obtained with the use of a Quantum Design SQUID magnetometer MPMS-XL. This magnetometer works between 1.8 K and 400 K for dc applied fields ranging from −7 T to 7 T. Measurements were performed on a polycrystalline sample of 6.9 mg and 6.7 mg for complexes 1 and 2, respectively. M versus H measurements have been performed at 100 K to check for the
presence of ferromagnetic impurities that has been found absent. The magnetic data were corrected for the sample holder and the diamagnetic contribution.
Single crystal X-ray diffraction measurements were performed at 173 K by using a Nonius Kappa CCD or a Bruker APEX-II CCD diffractometer with Mo-Kα
radiation. The crystal structures were solved by direct methods and refined by employing full-matrix least-squares on F2 (SHELXL-97). All nonhydrogen atoms were refined anisotropically, and the hydrogen atoms of organic ligands were introduced by using a riding model (SHELXL-97). CCDC deposition numbers 1559577 (for complex 1) and 1559576 (for complex 2) contain the supplementary crystallographic data for this paper. These data can be obtained free of charge from the Cambridge Crystallographic Data Center via https://www.ccdc.cam.ac.uk/structures/.
Ligand HL1. 2-pyridinamine (1.42 g, 15 mmol, 1 equiv.) in EtOH (50 mL) was added to a vigourously stirred solution of picolinaldehyde (1.61 g, 15 mmol, 1 equiv.) in EtOH (75 mL) at RT. Then, the resulting mixture was further stirred at this temperature for 1 h, before being refluxed for 3 h, and finally stirred overnight at RT. The resulting mixture was then evaporated under vacuum to yield a pale crystalline solid (99%). All analyses correspond to those described in the literature (see, e.g., [20] and references therein).
Complexes 1 and 2. To a 5-minute stirring solution of HL1 (366 mg, 2 mmol, 2 equiv.) in CH3CN (15 mL) we added targeted Mn(NO3)2·4H2O (250 mg, 1 mmol, 1 equiv.) or MnCl2·4H2O (200 mg, 1 mmol, 1 equiv.). The resulting brown solution was further stirred for 1 h at this temperature and filtrated over
cotton. Dark brown or green block shaped crystals
(20–40%) identified as mononuclear species, for complexes 1 and 2, respectively, were obtained by slow evaporation after a 1-to-3-day standing at RT (caps perforated with ca. 13-7 holes). KBr: [complex 1] 550 cm−1, 760 cm−1, 1,050 cm−1, 1,300 cm−1, 1,380 cm−1, 1,530 cm−1, 1,620 cm−1, 3,350 cm−1; [complex 2] 553 cm−1, 757 cm−1, 1,051 cm−1, 1,302 cm−1, 1,377 cm−1, 1,534 cm−1, 1,620 cm−1, 3,356 cm−1. Anal. Calcd for C22H20ClMnN6O2 (490.83): C, 53.83; H, 4.11; N, 17.12. Found: C, 53.91; H, 4.16.6; N, 17.4. Anal. Calcd for C22H24MnN7O7 (553.42): C, 47.74; H, 4.37; N, 17.72. Found: C, 47.43; H, 4.41; N, 17.6.
Two new discrete Mn complexes made of unexpected heteroleptic ligand have been synthesized and structurally characterized. A conspicuous feature of the structure of these cationic complexes [MnL22]+ is compression along the z-axis, resulting in an axially-compressed octahedral geometry.
The mixed-donor polydentate ligand employed in this study has favored this remarkable
Jahn-Teller distortion. These new compounds exemplify the conflict between stabilization from the Jahn-Teller effect and the stereochemical
requirements of the chelate ring system. Given the technique developed by Tregenna-Piggott, we believe that these systems could be good candidates for the prediction of the nature of the Jahn-Teller distortion in bioinorganic Mn(III) and copper(II) centers. These may interest others. Hence, the biological impact of complexes 1 and 2 may notably be assessed in relevance to: (i) their interaction with DNA (e.g., calf-thymus), (ii) their antimicrobial activity against microorganisms (e.g., Escherichia coli), and (iii) their affinity to bovine (BSA) and human (HSA) serum albumins, since the binding to these proteins which are involved in the transport of metal ions and compounds through the bloodstream may provide important information for the transportation of the compounds within the body, as a first means to potential applications
[21].
The author acknowledges the Institute für Anorganische Chemie (KIT, Karlsruhe, Germany)
for the provision of instrumentation on the X-ray diffractometer. Elodie Soehnlen, D. Seyller, C. Grau, and M. Schamberger—students
(tutorial project)—are thanked for the resynthesis of
the ligand. Dr. Valeriu Mereacre is gratefully thanked for the scientific discussions and careful reading of the manuscript.
The author declares that he has no conflict of interest.
- V. L. Pecoraro, Manganese Redox Enzymes, VCH Publishers, New York, 1992.
- G. Renger, Biological exploitation of solar energy by photosynthetic water splitting, Angew Chem Int Ed Engl, 26 (1987), 643-660.
- M. L. Ludwig, K. A. Pattridge, and W. C. Stallings, Manganese in Metabolism and Enzyme Function, Academic Press, New York, 1986.
- G. E. O. Borgstahl, H. E. Parge, M. J. Hickey, W. F. Beyer Jr., R. A. Hallewell, and J. A. Tainer, The structure of human mitochondrial manganese superoxide dismutase reveals a novel tetrameric interface of two 4-helix bundles, Cell, 71 (1992), 107-118.
- A. M. Ako, I. J. Hewitt, V. Mereacre, R. Clérac, W. Wernsdorfer, C. E. Anson, et al., A ferromagnetically coupled Mn19 aggregate with a record S=83/2 ground spin state, Angew Chem Int Ed Engl, 45 (2006), 4926-4929.
- D. C. Weatherburn, Manganese-containing enzymes and proteins, in Handbook on Metalloproteins, I. Bertini, A. Sigel, and H. Sigel, eds., Marcel Dekker, New York, 2001, 193-268.
- P. E. Siegbahn, Quantum chemical studies of manganese centers in biology, Curr Opin Chem Biol, 6 (2002), 227-235.
- C. R. Goldsmith, A. P. Cole, and T. D. P. Stack, C−H activation by a mononuclear manganese(III) hydroxide complex: synthesis and characterization of a manganese-lipoxygenase mimic?, J Am Chem Soc, 127 (2005), 9904-9912.
- H. Miyasaka, A. Saitoh, and S. Abe, Magnetic assemblies based on Mn(III) salen analogues, Coord Chem Rev, 251 (2007), 2622-2664.
- S. Ding, Y.-F. Ji, M.-Y. Kang, C.-F. Du, Z.-L. Liu, and C.-M. Liu, In situ synthesis of manganese(III) complexes under control: Crystal structure and magnetic properties, Inorg Chem Commun, 21 (2012), 96-99.
- M. S. Shongwe, S. K. Vandayar, M. A. Fernandes, H. M. Marques, M. J. Morris, and S. L. Heath, Manganese(III) in a pseudo-compressed mixed-donor octahedral environment: synthesis, X-ray crystal structure and physicochemical properties, Polyhedron, 20 (2001), 2195-2201.
- P. L. Tregenna-Piggott, Origin of compressed Jahn-Teller octahedra in sterically strained manganese(III) complexes, Inorg Chem, 47 (2008), 448-453.
- C. Mantel, A. K. Hassan, J. Pécaut, A. Deronzier, M. N. Collomb, and C. Duboc-Toia, A high-frequency and high-field EPR study of new azide and fluoride mononuclear Mn(III) complexes, J Am Chem Soc, 125 (2003), 12337-12344.
- M. U. Triller, D. Pursche, W. Y. Hsieh, V. L. Pecoraro, A. Rompel, and B. Krebs, Catalytic oxidation of 3,5-Di-tert-butylcatechol by a series of mononuclear manganese complexes: synthesis, structure, and kinetic investigation, Inorg Chem, 42 (2003), 6274-6283.
- Q. Scheifele, C. Riplinger, F. Neese, H. Weihe, A. L. Barra, F. Juranyi, et al., Spectroscopic and theoretical study of a mononuclear manganese(III) complex exhibiting a tetragonally compressed geometry, Inorg Chem, 47 (2008), 439-447.
- I. J. Hewitt, J. K. Tang, N. T. Madhu, R. Clérac, G. Buth, C. E. Anson, et al., A series of new structural models for the OEC in photosystem II, Chem Commun, (2006), 2650-2652.
- M. S. Shongwe, M. Mikuriya, R. Nukada, E. W. Ainscough, A. M. Brodie, and J. M. Waters, A series of heteroleptic complexes of the type fac-[MnIIIL2]-[H2L = derivatives of N-(2-hydroxybenzyl) glycine or N-(5-nitro-2-hydroxybenzyl) sarcosine] possessing unusual Mn(III) co-ordination spheres, Inorganica Chim Acta, 290 (1999), 228-236.
- G. Wu, I. J. Hewitt, S. Mameri, Y. Lan, R. Clérac, C. E. Anson, et al., Bifunctional ligand approach for constructing 3d-4f heterometallic clusters, Inorg Chem, 46 (2007), 7229-7231.
- C. Perthuisot, M. Fan, and W. D. Jones, Catalytic thermal C−H activation with manganese complexes: evidence for η2-H2 coordination in a neutral manganese complex and its role in C−H activation, Organometallics, 11 (1992), 3622-3629.
- A. Taywade, S. Chavan, A. Ulhe, and B. Berad, Unique CuI-pyridine based ligands catalytic systems for N-arylation of indoles and other heterocycles, Synth Commun, 48 (2018), 1443-1453.
- C. Tan, J. Liu, H. Li, W. Zheng, S. Shi, L. Chen, et al., Differences in structure, physiological stability, electrochemistry, cytotoxicity, DNA and protein binding properties between two Ru(III) complexes, J Inorg Biochem, 102 (2008), 347-358.
Copyright © 2019 Samir Mameri. This is an open access article distributed under the terms of the Creative Commons Attribution License, which permits unrestricted use, distribution, and reproduction in any medium, provided the original work is properly cited.